9. Osmoregulation
Water, and Regulation of the Animal’s Internal Environment
- 9.1 Introduction
- 9.2 Homeostasis of Internal Fluids
- 9.3 Waste Nitrogen, and its Effect on Water Balance
- 9.4 Osmotic Challenges in Animals
- 9.5 Osmoregulatory Organs
- 9.6 Malpighian Tubules
- 9.7 The Kidneys
- 9.8 Summary
9.1 Introduction
Water is an essential nutrient vital to all forms of life, even though it does not provide calories or organic nutrients. The main source of water in most animals is dietary intake from free water, as well as from water contained in solid foods. An additional source of water is metabolic oxidation, internal production of water by oxidizing hydrogen-containing molecules in reserve tissues and nutrients ingested with food. Metabolism in the mitochondria of cells produces about 100 grams of water per 100 grams of fat, 42 grams of water per 100 grams of protein and 60 grams of water per 100 grams of carbohydrate; but the overall need for water in most animals exceeds their ability to produce it. Some animals (xerocoles1) that live in dry environments rely mostly on metabolic water (Figure 9.1, below). Birds on non-stop migratory flights also rely heavily on metabolic water (Figure 9.2, below). In humans, only 8% to 10% of water is from metabolism.
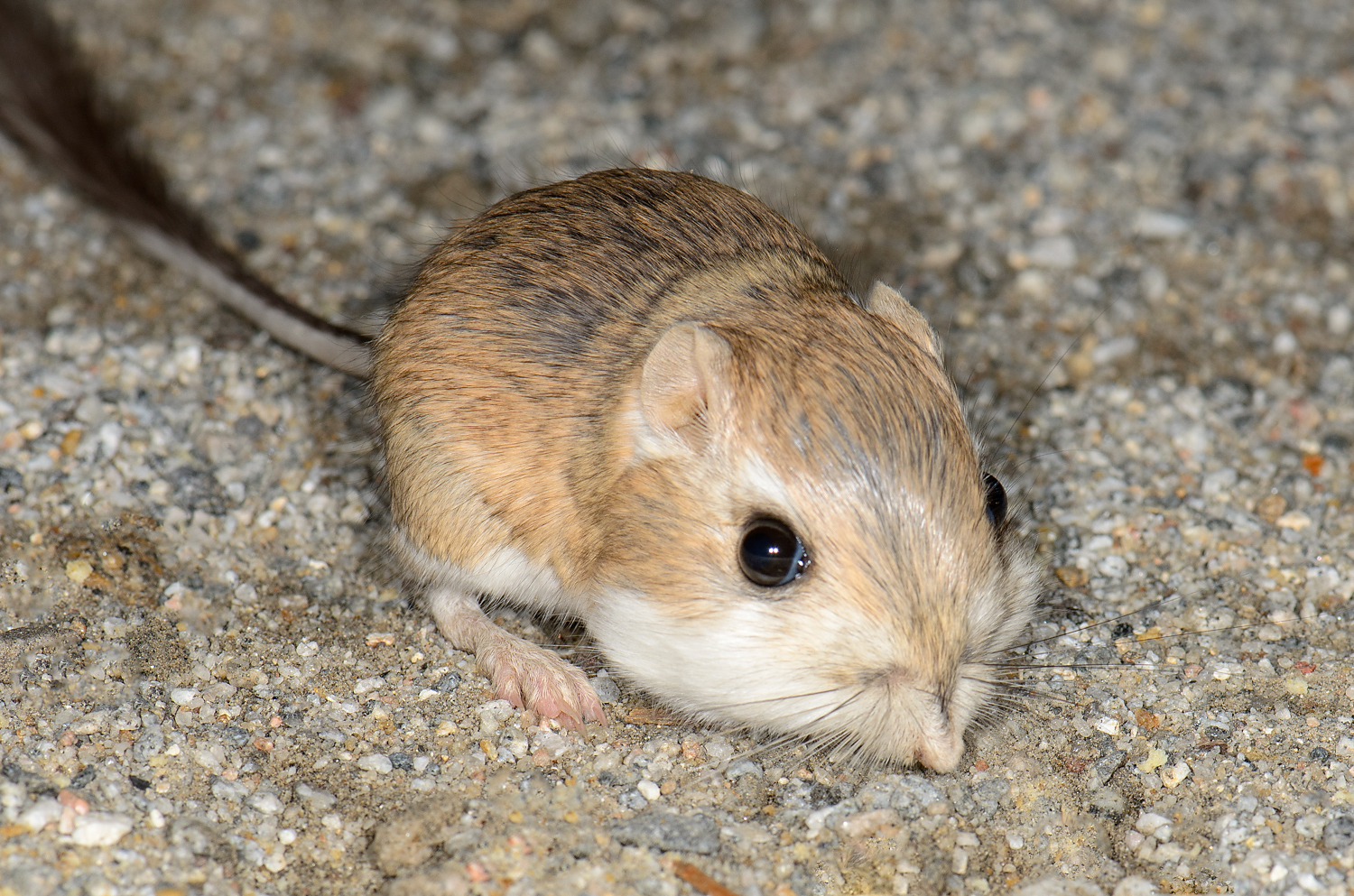

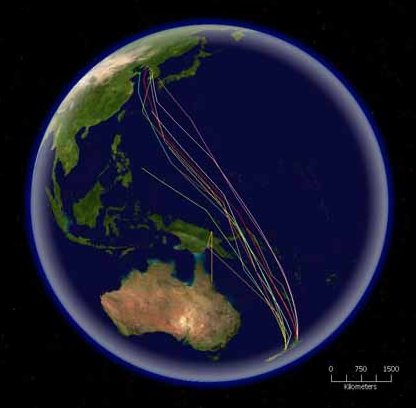
These water–based internal body fluids contain an abundance of solutes, both small molecules, and macromolecules. Small molecule (low molecular mass) solutes include the ions sodium (Na+), chloride (Cl–), potassium (K+), magnesium (Mg++), calcium (Ca++), and phosphate (PO3-4). Among macromolecules (high molecular mass), proteins are the most abundant. Homeostasis maintains a stable composition of these body fluids essential to the normal functioning of all cells and tissues.
As a universal solvent, water plays an essential role in the composition of the internal body fluids, as it is required for maintaining an appropriate environment for the metabolic reactions that occur in the water based fluid of the cellular environment. Water fills the spaces in and between cells and helps to form the three-dimensional structure of large molecules such as proteins, nucleic acids, and carbohydrates, and also helps form the structure of biological membranes.
Osmosis is the bulk movement of solvent across a semipermeable membrane by passive diffusion. Solvent tends to accumulate on the side with higher solute concentration.
Osmotic pressure is the amount of hydrostatic pressure that would be needed to cancel out osmosis. Osmotic pressure is one of the colligative properties of solutions, which means that the pressure depends on the molar concentration of the solvent but not its chemical identity.
Water enters and leaves the cell by osmosis; from a compartment that has a lower solute concentration to a compartment that has a greater solute concentration. The solvent moves by osmosis until solute concentrations are equal on both sides of the compartment. The side with higher concentration of solutes increases in volume, therefore osmosis exerts pressure that can do work. Thus, osmotic pressure is the external force required to block solvent movement across the membrane exerted by a greater concentration of solutes.
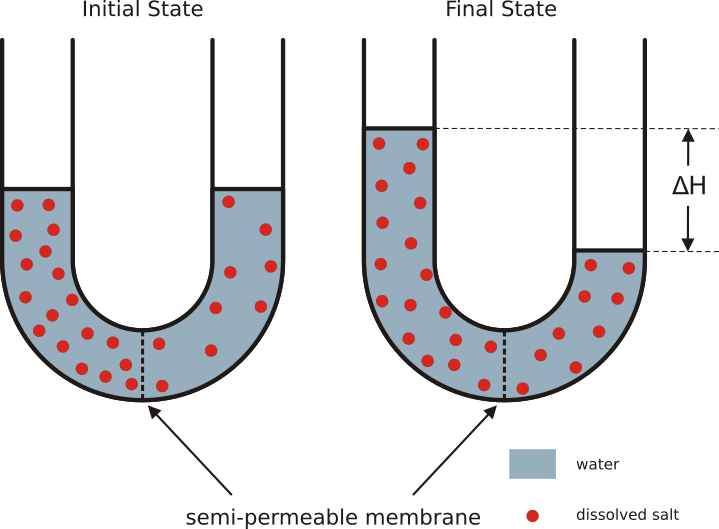
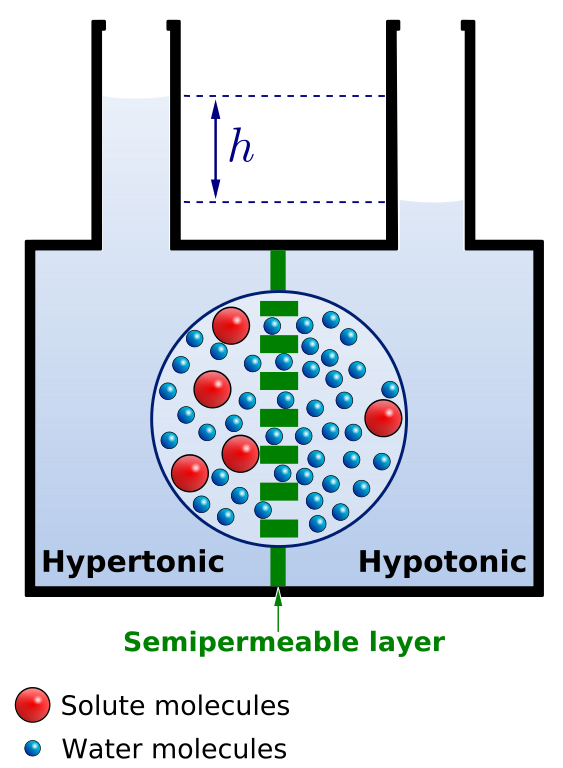
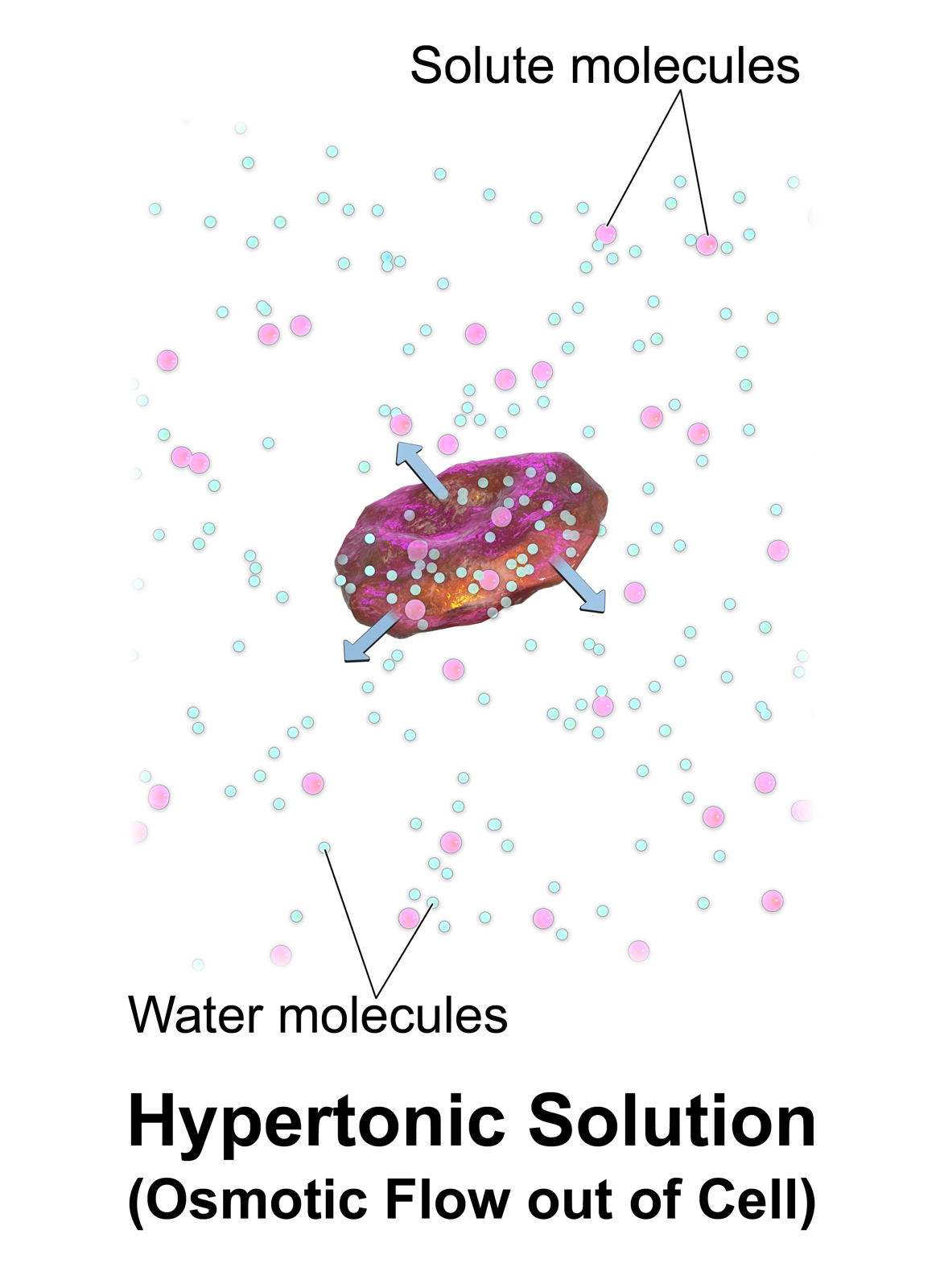
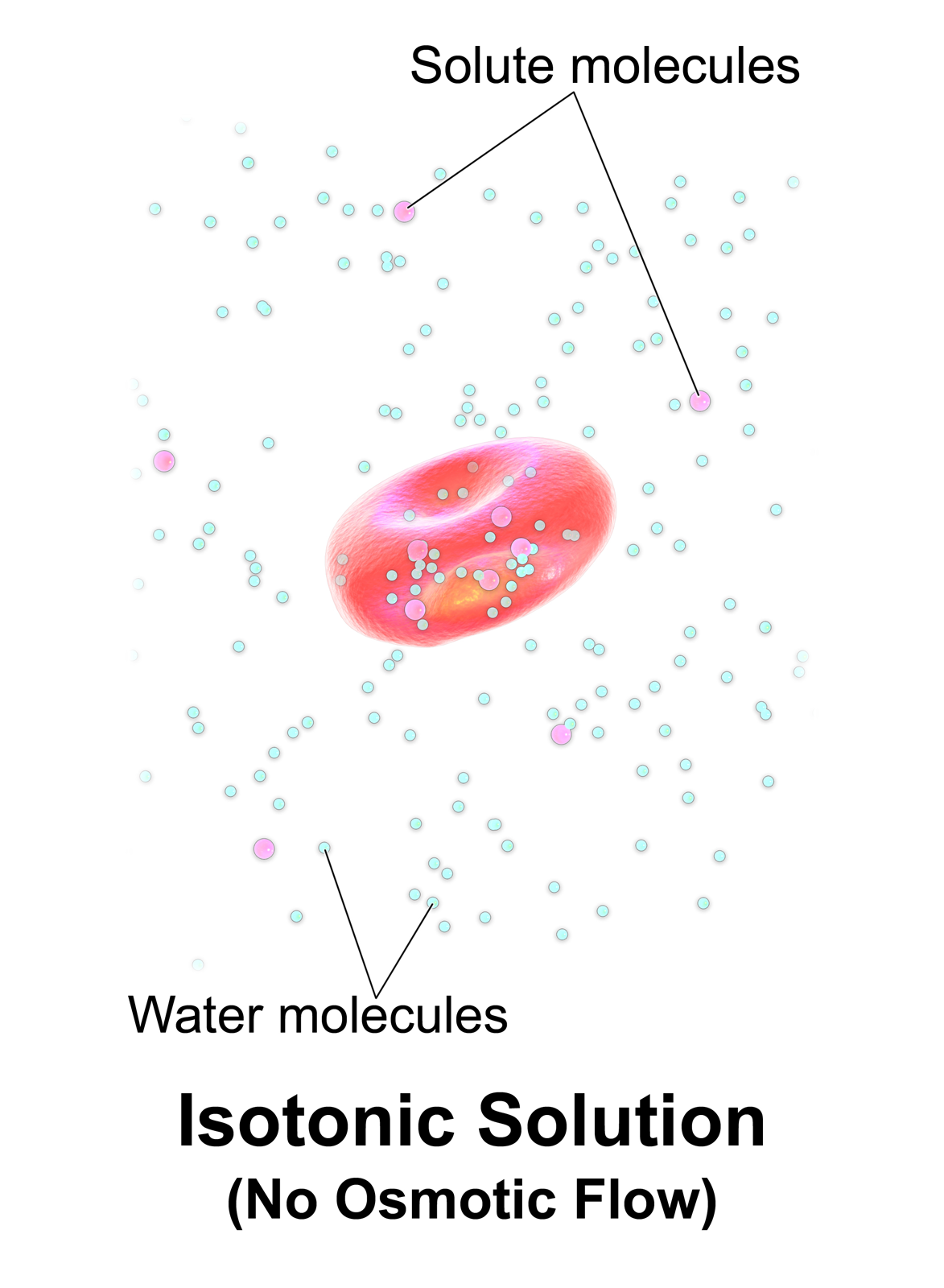
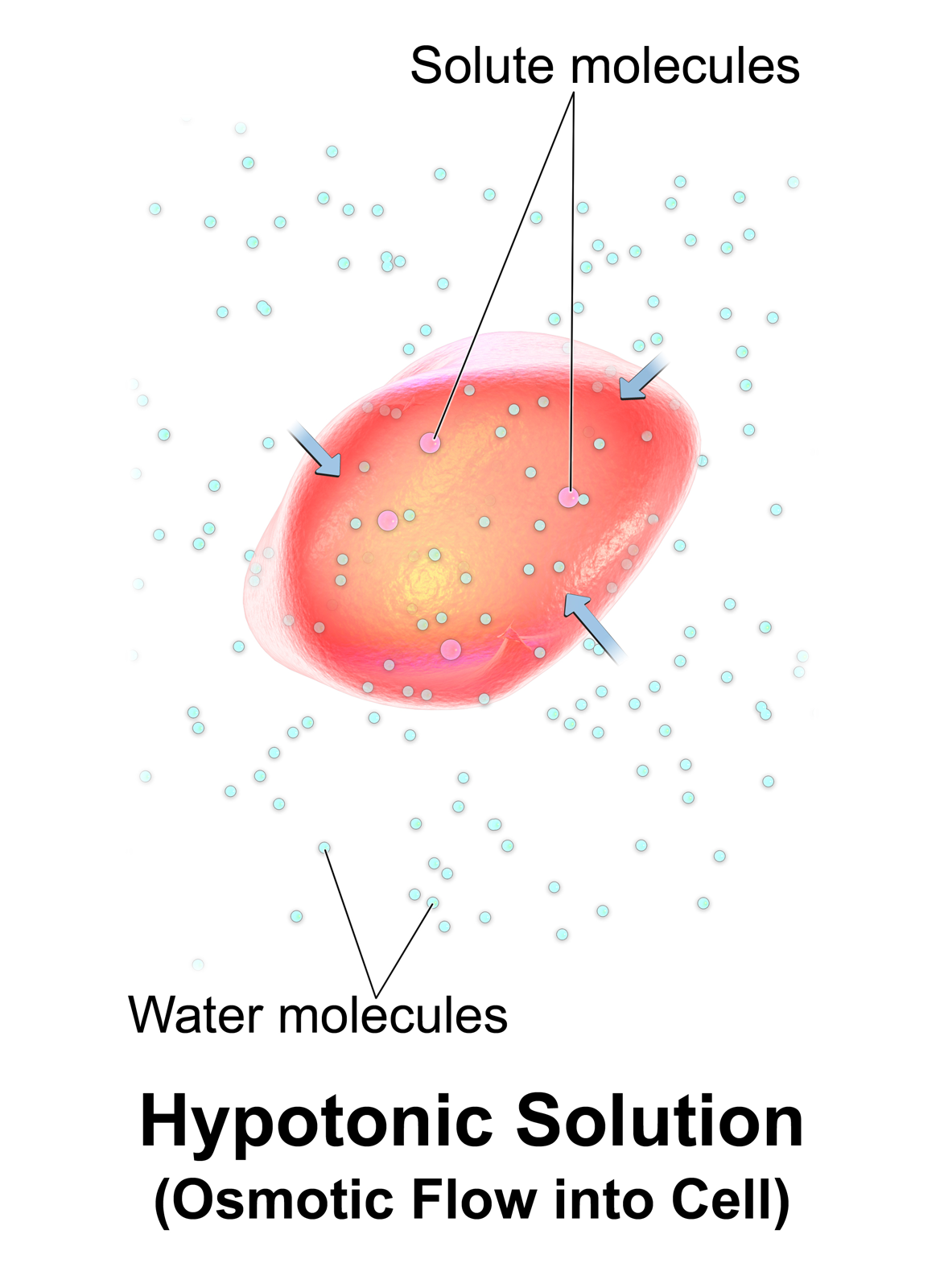


It’s important to keep in mind that cells don’t have any way to actively transport water across membranes. There are plenty of known solute pumps that expend energy to pump solutes across a membrane, but no known transmembrane water pumps. The only way cells can move water in one direction across membranes is to pump solute, and allow water to passively follow the solute by osmosis. Osmosis arises from the purely random motion of water molecules – diffusion – but cells can harness it to move large amounts of water in one direction by actively pumping solutes across membranes. This concept is central to understanding how the animal body and its organs manage water balance.
Some terms that can be confusing: Molarity, osmolarity, and osmolality are three different measures of concentration: Molarity is the concentration of a dissolved chemical, the amount of the compound per unit volume of solution, usually expressed as moles per litre (molar, M) or millimoles per litre (millimolar, mM). Osmolarity is the concentration of solute particles, the number of particles (freely diffusing atoms or molecules) per unit volume of solution, usually expressed as osmoles per litre (osmolar, Osm/L) or milliosmoles per litre (milliosmolar, mOsm/L). This contrasts with molarity, which is based on the solute’s chemical formula, without regard to how many solute particles are bouncing around in solution.
For example, a 100 mM solution of table salt (NaCl) in water is almost 200 mOsm/L, because the NaCl dissociates into separate Na+ and Cl– ions in solution. In physiology, osmosis is important because it determines how much water crosses membranes, in which direction – and it’s the number of solute particles that’s relevant for osmosis, not their chemical identity.
Which brings us to osmolality. Like osmolarity, osmolality is the concentration of solute particles, but osmolality is calculated in a different way: the number of solute particles per unit mass of solvent, usually expressed as osmoles per kilogramme (Osm/kg) or milliosmoles per kilogramme (mOsm/kg). This contrasts with osmolarity, which is per unit volume of solution. Compared with osmolarity, omsolality is more directly related to osmotic pressure, because it has a close mathematical relationship with the number of solvent molecules colliding with a surface: Two solutions with equal osmolality will have equal osmotic pressure. Nevertheless, for practical purposes, there isn’t much difference between osmolarity and osmolality of aqueous solutions when solute concentrations are in the range we see most frequently in the animal body. When discussing physiology, people often use osmolarity and osmolality interchangeably because the difference in values tends to be less than the measurement error: A 200 mOsm/L NaCl solution is roughly 201 mOsm/kg.
Water moves constantly in the body through a continuous cycle of metabolism, evaporation, sweating, reabsorption and elimination through the urinary and digestive systems, but only the osmoregulatory organs – Malpighian tubules in insects and kidneys in vertebrates – regulate water loss specifically to maintain osmotic pressure and water balance.
Specific heat capacity is defined as the amount of heat that one gram of a substance must absorb or lose to change its temperature by one degree Celsius (°C). Water has one of the highest specific heat capacities of any liquid, equal to 4.186 joule/gram °C. This provides the definition of the calorie: The specific heat capacity of water is 1 calorie/gram °C. The presence of hydrogen bonds between water molecules is the main reason why water has the highest specific heat capacity of any commonly occurring substance. Breaking of a hydrogen bond absorbs some of the kinetic energy of the molecules (the two water molecules slow down) and stores it as potential energy (the two water molecules accelerate when they form a hydrogen bond again). High specific heat capacity is an advantage for organisms, as it helps buffer the body against changes in temperature.

Because of water’s high specific heat capacity, water protects the body from rapid changes in temperature. The body being 70% water, body temperature doesn’t change much compared with the amount of heat the body absorbs or releases when ambient temperatures are high or low. Thus, regulation of water contents and the concentrations of dissolved solutes – osmoregulation – and maintaining an adequate body temperature – thermoregulation – are critical mechanisms for homeostasis.
Osmoregulation involves controlling an adequate equilibrium between water loss and gain, while thermoregulation involves controlling body temperature at adequate levels for optimal enzyme activity in all metabolic reactions. Optimal body temperature is required to maintain the three dimensional structure of proteins and enzyme activity compatible with body needs. Low body temperatures reduce enzyme activity, while temperatures beyond optimal levels can cause protein unfolding and losing their three dimensional structure, and therefore its activity. Thus, animals exposed to habitats with extreme temperature variations adapted to it by expressing protein isoforms with a wider range of optimal enzymatic activities.
In addition to osmoregulation and thermoregulation, there is a need for the animal body to control concentrations of inorganic ions, and eliminate wastes such as water-soluble by-products of metabolism, nitrogen wastes, toxins from food, and hormones. Thus, osmotic pressure, body temperature, and levels of metabolic by-products are closely linked physiological variables that are integrated by an interaction between neural and humoral regulatory mechanisms (described in Chapter 12) essential for maintaining body homeostasis. In this chapter, we describe the role of the main osmoregulatory organs, while we describe thermoregulation in Chapter 13.
9.2 Homeostasis of Internal Fluids
Four groups of organs are directly responsible for regulating solute concentrations, controlling water balance, and removing wastes. They include:
- Respiratory systems: The lungs and gills dispose of CO2. In aquatic animals, gills remove ammonia (NH3) and excess bicarbonate ions (HCO3–) from the blood.
- Digestive system: The gut eliminates water-insoluble waste products such as undigested fibre and bacteria by producing feces. The digestive system of vertebrates also excretes the waste molecule bilirubin, a product of the breakdown of the heme2 moiety of red blood cells’ hemoglobin. The liver captures bilirubin and its precursor biliverdin from the blood plasma, makes the bilirubin water-soluble by attaching glucuronyl groups, and secretes the bilirubin conjugate into the bile that flows into the duodenum. In addition, the epithelia of the gastrointestinal tract are involved in ion and water regulation.
- Skin and epithelial glands: can excrete water-soluble organic wastes with the sweat fluids. In many amphibians, the skin regulates salt and water uptake. In vertebrates, an important amount of water can be lost or eliminated by insensible loss by sweating through the skin.
- Renal organs (Latin, renalis: kidney): kidneys in vertebrates, nephridia in many invertebrates, and Malpighian tubules in insects, clean body fluids and regulate water, ions, and many organic compounds. Renal organs selectively reabsorb or secrete these and other molecules.
9.3 Waste Nitrogen, and its Effect on Water Balance
Metabolism of nutrients for energy generates CO2 and water. In the case of lipids and carbohydrates, CO2 and water are the only products of cellular respiration. However, the same isn’t true of proteins and nucleic acids when they are degraded for use as an energy source, because they contain a large amount of nitrogen. Proteins are hydrolysed into amino acids. Amino acids may be reused to make new proteins, or be converted into osmolytes3 that contribute to osmoregulation, as in several marine animals (described below). Any nitrogen in excess of the animal’s immediate needs have to be converted into nitrogenous wastes. The simplest – but most toxic – nitrogenous waste is ammonia (NH3). NH3 binds protons and becomes the ammonium ion (NH4+). There are two main ways that ammonium poisons cells:
- Ammonium is a strong base that can alter the internal acid-base equilibrium, and
- ammonium affects membrane ion transport by replacing K+ in the ion pumping catalyzed by the Na+/K+ ATPase (See Chapter 5).
Therefore, animals must rapidly dispose of all NH3 and NH4+ freed by breakdown and metabolism of protein and nucleic acid. either by dilution and excretion, or by converting them into less toxic forms, such as urea, and uric acid in its salt form, urate.
Thus, three different modes of nitrogen elimination can be distinguished in animals: ammonotely, ureotely, and uricotely.
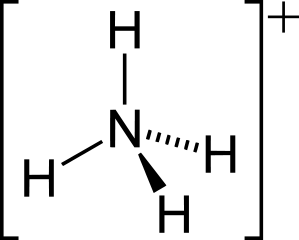
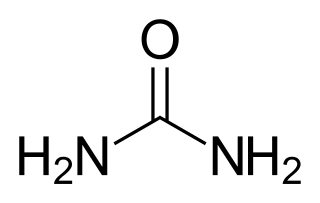
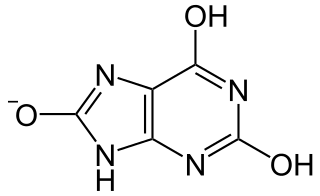
Ammonotely – Most aquatic animals, including most bony fishes, larval amphibians, and most invertebrates dispose of excess nitrogen as ammonia (NH3). Because ammonia is highly soluble in water, these animals can get rid of ammonia by diffusion through their respiratory surfaces into their watery environment. As long as the habitat isn’t overcrowded, the large amount of water in the environment dilutes the ammonia below toxic levels. Even small amounts of ammonia in the environment will cause sickness and death in most aquatic animals, so dilution is important. Animals that produce ammonia waste are called ammonotelic animals (Greek, télos: end or product).
Ureotely – Terrestrial animals cannot dilute NH3, therefore they must convert it into urea, for which they are called ureotelic animals. The reaction for producing urea requires an additional expenditure of energy compared with NH3 (4 moles ATP per mole of NH3). But urea is 10 to 100 times less toxic than NH3. Hence, urea can be accumulated at much higher concentrations. Urea is produced from two nitrogen ions, giving the additional benefit of removing two nitrogen atoms per molecule. Overall, it takes less water to excrete nitrogen as urea than as ammonia. Amphibians excrete NH3 via their gills during their aquatic larval stages, but as semiaquatic adults switch their nitrogen metabolism to allow the liver to convert NH3 into urea that is excreted through the kidneys.
In most vertebrates, urea is generated via the ornithine-urea cycle (OUC), in which the amino acid aspartic acid and HCO3– are metabolized to produce urea, using ATP as a source of energy, although some bony fishes produce urea through the uricolytic pathway.

Urea is toxic at high concentrations (greater than 100 mM) because it destabilises the three-dimensional folding of proteins. However, marine chondrichthyan fishes (cartilaginous fish, for example sharks) and coelacanths (an unusual kind of “living fossil” bony fish) are able to accumulate urea to much higher levels (up to 400 mM). The kidneys of chondrichthyan fishes and coelacanths counteract the destabilizing effect of urea by producing another osmolyte, methylamine trimethylamine N-oxide (TMAO), which protects protein folding. TMAO stabilizes proteins to withstand high concentrations of urea in chondrichthyans and coelacanths. Thus in these marine species, special solutes, in this case urea and TMAO, control osmotic pressure.
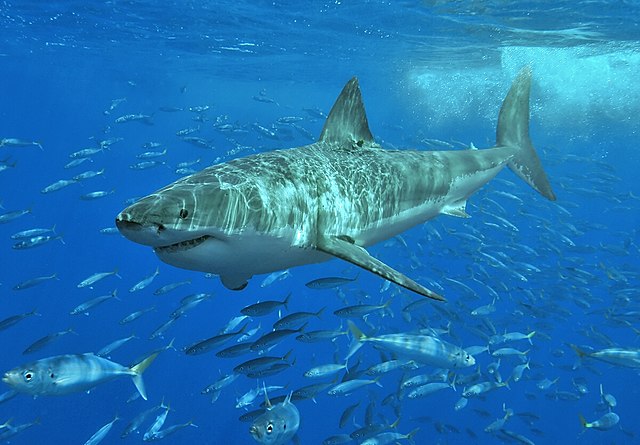
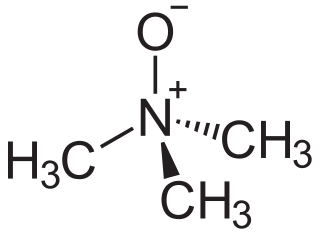
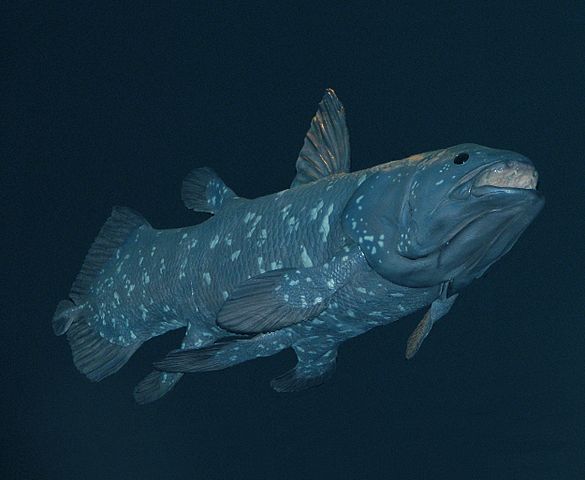
Cows aren’t known for eating a lot of meat. Their diet is carbon-rich, but extremely nitrogen-poor, mostly consisting of cellulose plant walls that the cow relies on her gut microbes to digest for her. So how does a cow supply her gut microbes with enough nitrogen to make proteins? Recycling.
In ruminants, urea is secreted in large amounts through the salivary glands. Urea-rich saliva enters the rumen where urease from microorganisms convert it back into NH3. Rumen microorganisms use NH3 for the synthesis of their own proteins, which are subsequently digested in the posterior chambers of the ruminant’s digestive system, releasing amino acids that the gut can absorb for re-use (see Chapter 8, on digestion). A general principle: Animals that rely on a symbiont as an energy source usually will have evolved a specific way to supply the symbiont with nitrogen.
Uricotely – Insects, most reptiles, birds, tree frogs, and desert toads dispose of excess nitrogen by producing uric acid. They are termed uricotelic animals, as the end product of amino acid and nucleic acid metabolism is uric acid. Production of uric acid is energetically more expensive (12 or more ATPs mole) than urea production, but it removes four nitrogens per molecule. Uricotely has a special advantage for animals that have limited access to external water: Uric acid dissolves poorly in water, allowing it to be excreted as solid crystals. It takes at least 50 times less water to excrete nitrogen as uric acid than as ammonia. In insects, most reptiles, and most birds, uric acid is excreted into the hindgut, eliminated along with feces as a semi-solid waste. Although the process of producing uric acid is energetically more expensive, it permits a more efficient use of water.
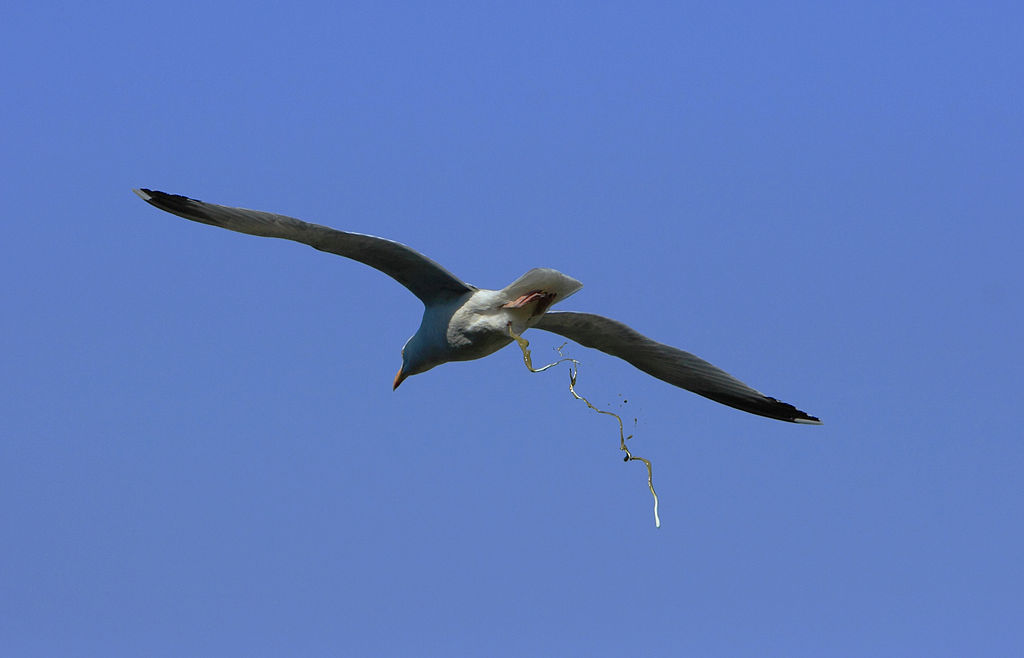
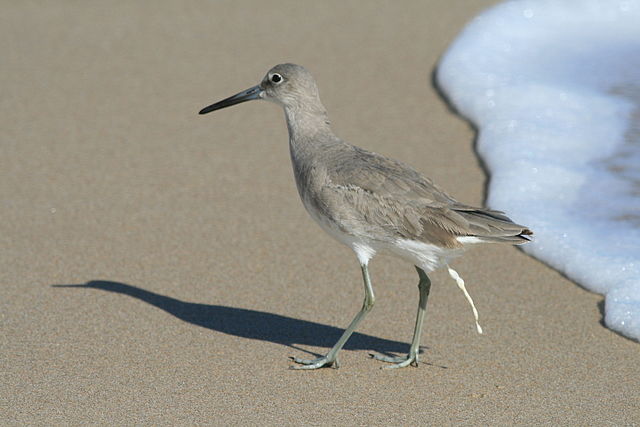
Uric acid’s insolubility in water is also useful in one situation where the animal cannot eliminate wastes at all: An embryo in an egg with an impermeable shell can’t excrete nitrogenous wastes to the environment, so nitrogenous waste has to build up somewhere inside the shell. Embryos of reptiles, birds, and monotremes (egg-laying mammals) have a special organ called the allantois that collects nitrogenous waste to safely store it within the egg. Uric acid excretion is indispensible if the embryo produces a large amount of nitrogen waste.
In general, animals adapted their nitrogen waste disposal mechanisms to the habitat and the availability of water in their environment. Although there are many exceptions, most aquatic animals get rid of nitrogen wastes by excreting ammonia, while most animals that live in dry habitats excrete either urea or uric acid.

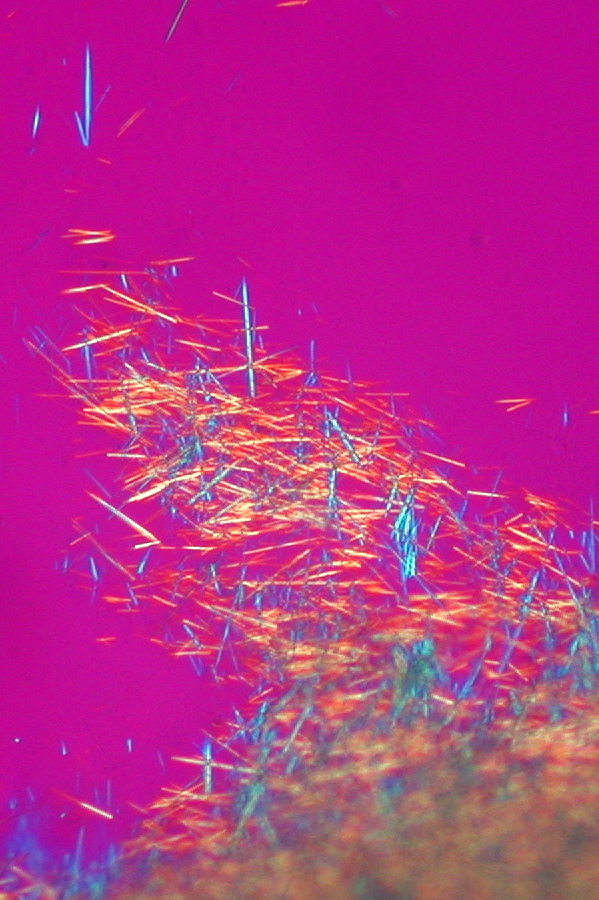
Why do humans get gout? Ureotelic animals – animals that excrete urea as their main nitrogenous waste – also produce a small amount of uric acid. The reason why we generate a little bit of uric acid is that it’s an intermediate in the catabolism of nucleic acids, specifically the bases adenine and guanine. In the pH of animal body fluids, uric acid forms ions and salts known as urates, which do not dissolve well in water. Most living things have the enzyme uricase (uric acid oxidase), which converts uric acid into a more water-soluble chemical. Apes are the exception. Apes – humans included – lack uricase, therefore we cannot manage high amounts of urates. In healthy individuals who do not ingest excessive amounts of alcohol or meat, the kidneys rapidly remove the small amount of urate and dispose of it as a normal component of urine. When the body produces too much uric acid, or if the kidneys malfunction, urates build up in joints or surrounding tissues. Eventually, this buildup leads to the painful arthritis known as gout.
9.4 Osmotic Challenges in Animals
Through the entire course of evolution, animals responded to a variety of osmotic challenges by adapting osmoregulatory organs to the conditions of their surrounding environment. Either in aquatic or terrestrial habitats animals needed to maintain proper solute concentrations and an efficient means of removal of metabolic wastes to maintain homeostasis. Four main osmotic environmental challenges for animals can be distinguished:
- Aquatic habitats with low solute concentrations, such as fish in fresh water environments of rivers, lakes and ponds;
- Aquatic habitats with high solute concentrations, such as seawater fish, as well as marine birds whose only source of water is seawater;
- Terrestrial habitats with free access to water; and
- Terrestrial habitats with limited access to water, such as in dry areas.
Changes within each of these four types of environment pose an osmotic challenge as well. Strategies to survive these changes can be divided into two broad categories: Osmoconformers are animals that evolved to change their internal osmotic pressure in response to their environment, while osmoregulators are animals that evolved to keep their internal osmotic pressure constant despite changes in the environment. The two groups overlap, of course, because many animals use both strategies depending on the circumstances.
- Osmoconformers are organisms that adapted essential cell functions to an internal media that resembles the osmotic pressure of their habitat. Most osmoconformers are aquatic, living in saltwater (marine) or brackish water. Although the internal osmolarity of osmocomformers is isosmotic to the external environment, the ionic composition is not necessarily the same. The solute composition of the internal fluids often differs greatly from the environment, but internal fluids remain isosmotic with the environment. Remember that osmotic pressure is a colligative property of solutions: The chemical nature of the solutes has no effect on osmosis, only the concentration of solute is important for osmosis. An example of different ionic composition in an isosmotic internal environment is found in the marine chondrichthyan fishes and coelacanths that accumulate urea and TMAO as major osmolytes.
- The main advantage of osmoconformation is that the organism does not expend too much energy to regulate osmotic gradients, although a small amount of energy is used in ion transport in order to ensure that the proper ions are present. The group of osmoconformers includes most marine invertebrates such as echinoderms, mussels, marine crabs, lobsters, jellyfish, and scallops.
- Depending on the capacity to resist external omolarities changes, osmoconformers can be classified as euryhaline and stenohaline (Greek, eurus: wide; steno: narrow; and haline: salt). Euryhaline osmoconformers are adapted to resist a wide range of osmotic changes such as fresh water, brackish4 water or salt water. Euryhaline organisms are commonly found in habitats such as estuaries where river waters mix with seawater and therefore salinity changes continuously. Salmon and eels are particular examples of euryhaline species, because as part of their life cycle they migrate between freshwater and marine environments. Stenohaline species only resist a narrow range of salinity. Most freshwater organisms are stenohaline as they will not survive in seawater. On the other hand most marine organisms are also stenohaline, but for the opposite reason, since they can’t survive fresh water environments.
- Osmoregulators include most vertebrates, which are able to maintain body fluids and solute concentrations within narrow ranges, regardless of the concentrations of solutes in their environment. This is an energetically expensive strategy. To maintain constant osmolarity and constant ion composition, osmoregulators need to expend a large amount of energy.
9.5 Osmoregulatory Organs
Although simple aquatic organisms do not have specialized osmoregulatory organs, some species such as sponges, cnidarians, and echinoderms dispose of soluble wastes by diffusion and through membrane transporters. Fresh water sponges have specialized vesicles for removal of excess water. In larger aquatic animals, the osmoregulatory systems evolved into more specialized tissues and complex organs, such as epithelial surfaces of skin, gills, and hindguts. Increased body size and varying environments pressured animals to develop organs with tubular structures lined with epithelia specialized for extracting and selectively retaining useful solutes, removing soluble wastes and excess inorganic ions, and balancing water volume. Thus, a variety of structures have evolved that enable animals to cleanse their body fluids and regulate water balance.
Many invertebrates have organs called nephridia (singular nephridium; Greek, nephron: kidney) which play a function similar to the vertebrate kidney in cleansing body fluids. Two basic types of nephridia are described:
- the protonephridium (Greek, prōto: first)
- the metanephridium (Greek, meta: after)
Other animals have excretory tubules, too, but they differ in important ways from nephridia:
- the Malpighian tubule in insects
- the nephron in vertebrates
The protonephridium is one of the simplest body fluid-cleansing organs found in animals. It consists of a series of branched, dead-end tubules. The closed end of each tubule consists of a single cell that has a large, lumen-facing tuft of cilia. The beating tuft of cilia looks like a flickering flame, so this cell is termed a flame cell. In some species, this cell has a flagellum instead, so it’s called a solenocyte (Greek, sōlḗn: pipe). In both cases, the beating of the cilia or the flagellum creates a negative pressure that draws body fluid into the tubule through slit-like openings surrounding the flame cell or solenocyte, and a positive pressure that pushes the filtered body fluid down the tubule. The slits are covered by a basement membrane: The gaps around the glycoproteins and proteoglycans of the extracellular matrix are large enough to let small molecules to pass, but leave large proteins retained within the animal body. The tubule cells reabsorb any needed solutes and water from the primary filtrate, and secrete soluble wastes before the urine is eliminated through small openings in the body wall called nephridiopores. In many animals with protonephridia, part of the tubule is lined with cilia that help propel the urine away from the flame cell towards the nephridiopore. Freshwater flatworms face the problem of excess water absorbed by osmosis through the skin, so the urine of their protonephridia is hypo-osmotic compared with the rest of the body fluids.
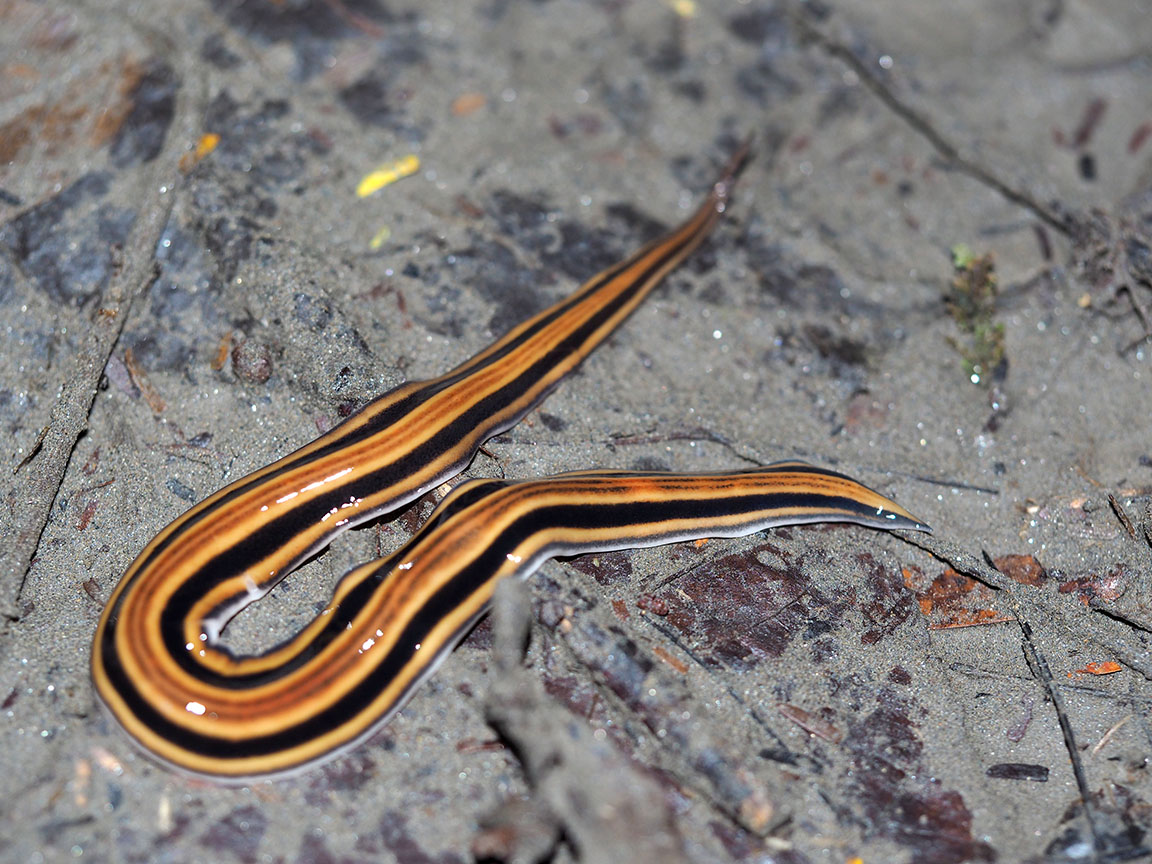
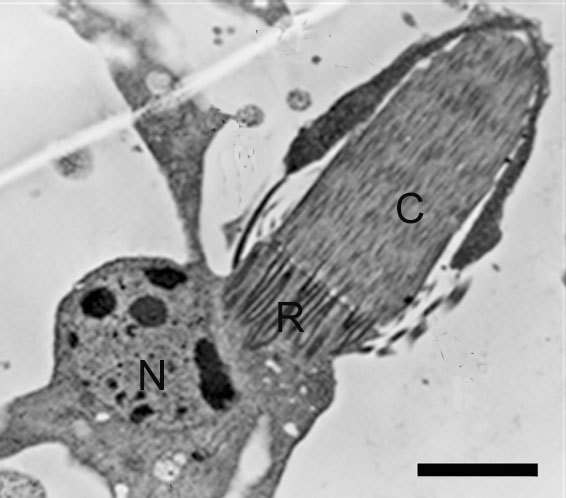
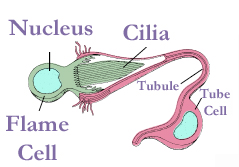
The metanepridia are a paired tubular system located in each body segment of annelids, for example, the earthworm. The defining feature of protonephridia is that they direct perform ultrafiltration of hemolymph. By contrast with protonephridia, metanephridia act on body fluids that have already been filtered elsewhere in the body. For example, the metanephridium of earthworms has ciliated opening in the shape of a funnel, termed the nephrostome. The nephrostome is located in the coelomic space, and takes up coelomic fluid, and connects to a folded duct in an excretory gland, leading to an opening – a nephridiopore – to the exterior. Primary urine – coelomic fluid in the case of the earthworm – is produced by filtration of blood, which is modified into secondary urine through selective reabsorption of sodium, chloride, and other solutes by active transport in the tubes that extend from the nephrostome.
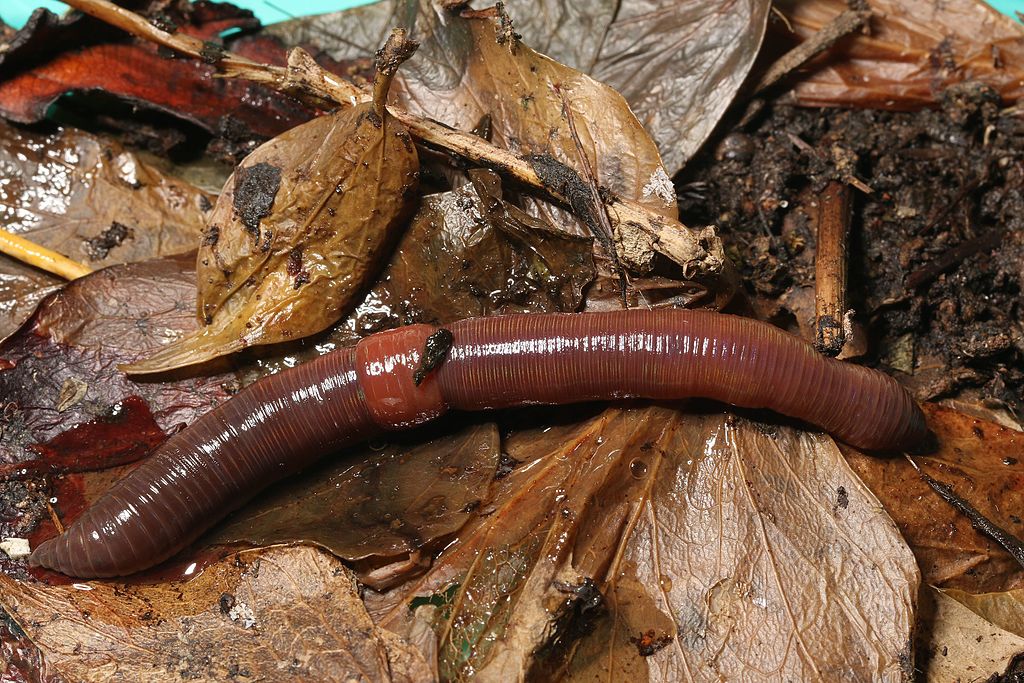
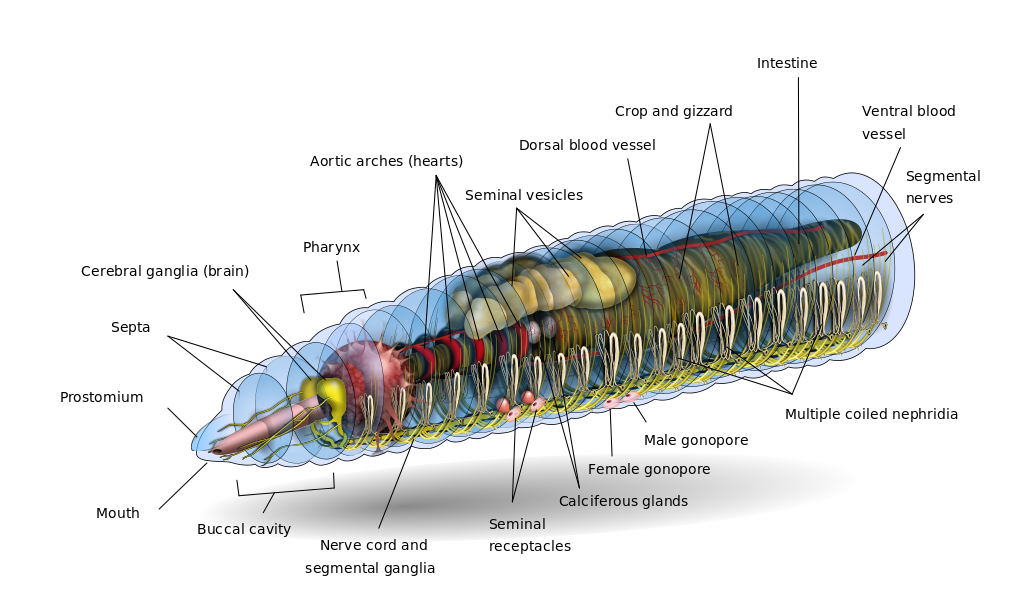


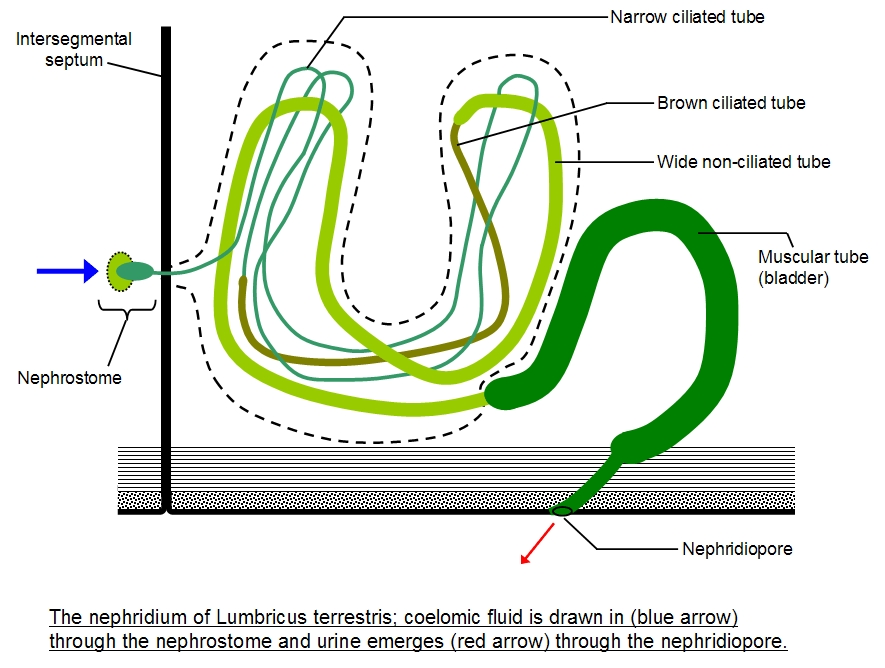
Diagrams: left, KD Schroeder, https://commons.wikimedia.org/wiki/File:Annelid_redone_w_white_background.svg CC BY-SA 4.0, 2016; right: Cronodon / BotRejectsInc, http://cronodon.com/BioTech/Earthworm_excretion.html CC BY-NC, 2007.
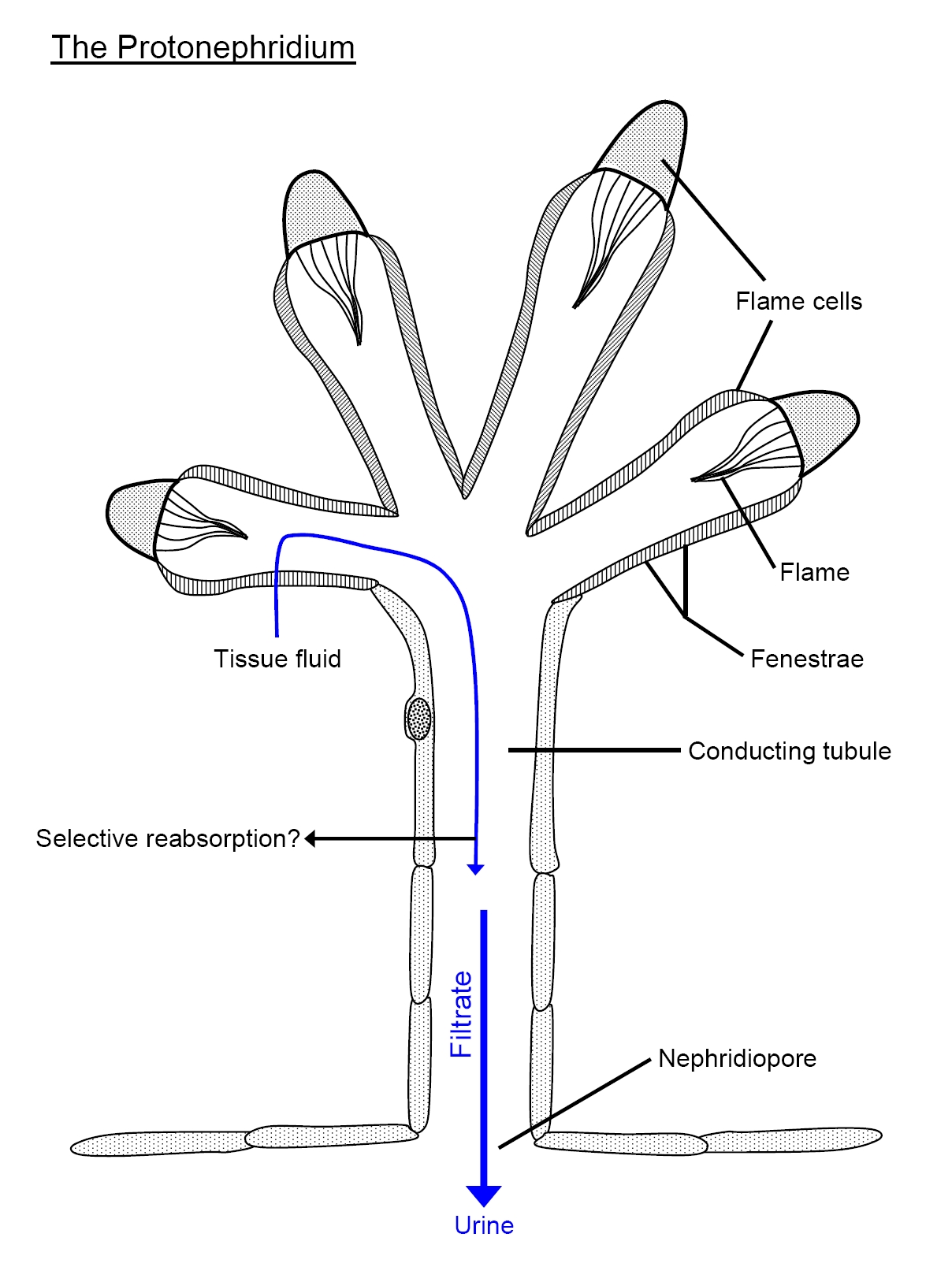
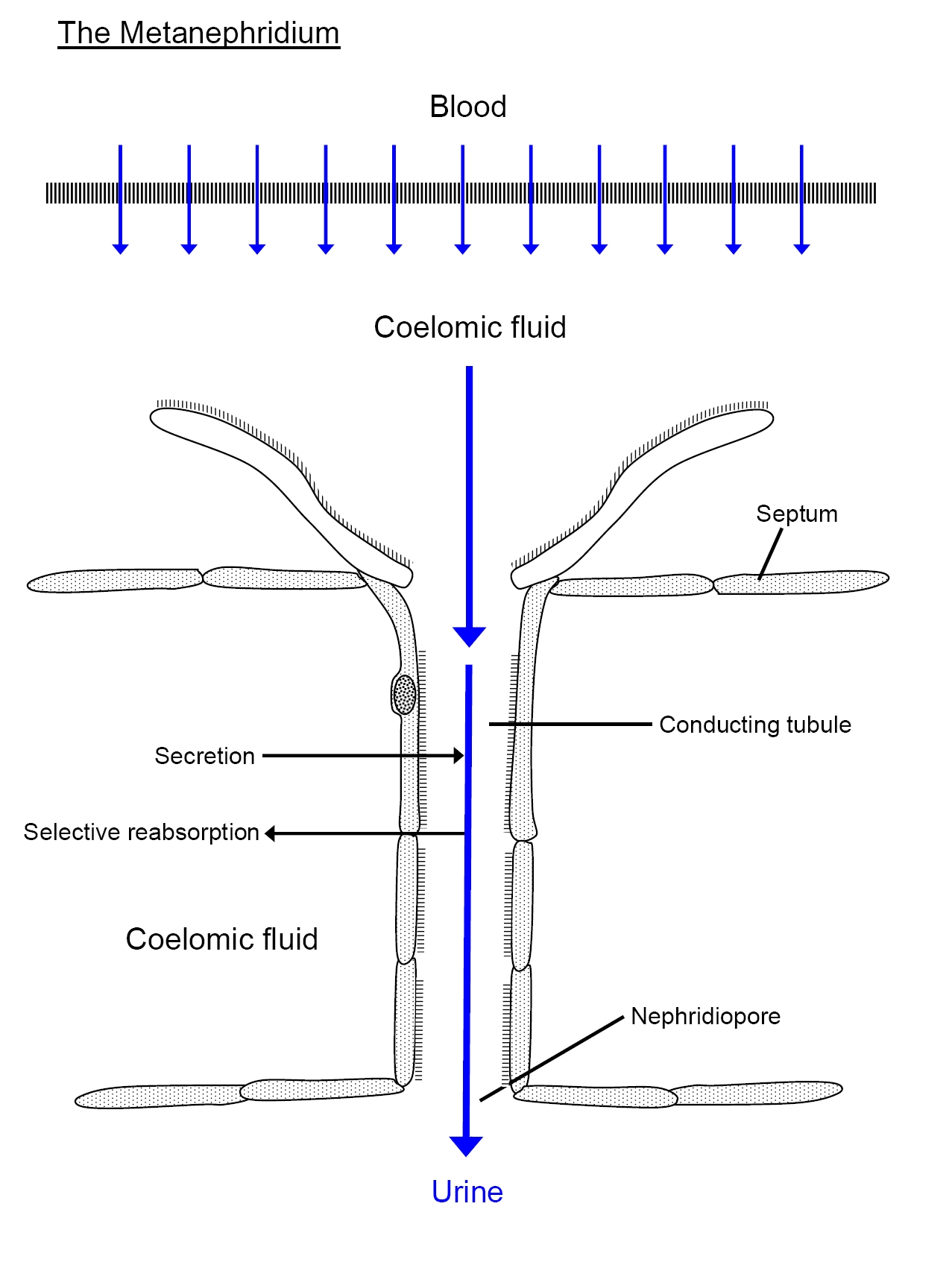
You’ll notice some similarities between excretory systems in very different animals, even when those excretory systems have evolved independently. As always, form follows function. Regardless of the species, the function of the osmoregulatory organs is to regulate the composition of the extracellular fluid and thereby to provide a nearly constant environment in which cells can function normally.
9.6 Malpighian Tubules
In most insects, slender tubules branching from the gut are the main organ cleansing the hemolymph. Called Malpighian tubules, these tubules are narrow ducts whose walls are one cell layer in thickness, and are blind-ended extensions from the digestive tract: One end of each Malphigian tubule empties into the gut at the junction between the midgut and hindgut, while the far end is closed off.
A salient feature of the Malpighian tubules is that they dispose of wastes by secretion only, not filtration. This contrasts with protonephridia and metanephridia of other invertebrates, and nephrons of vertebrates, which you’ll learn about later in this chapter.

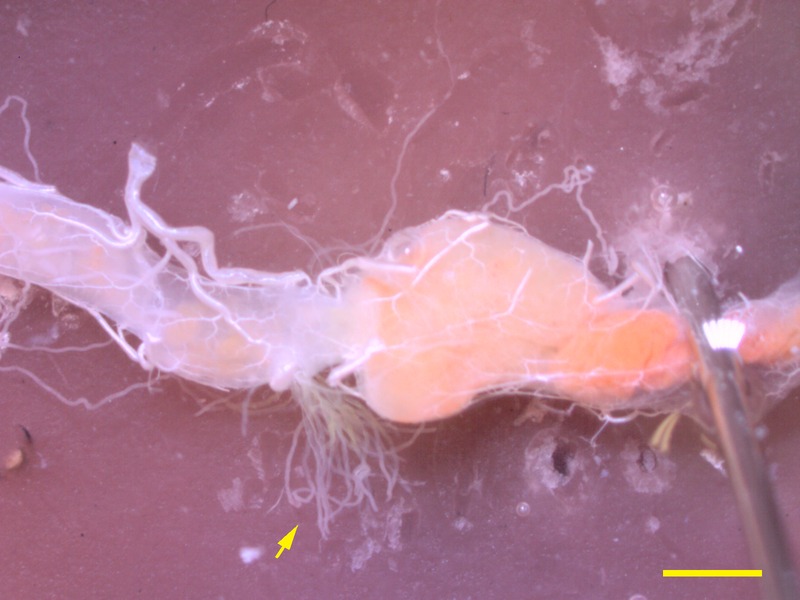


Insects secrete K+ (and often Na+) into the lumen of the Malpighian tubule. The middle segment of these tubules is rich in a vacuolar-type H+-ATPase (V-ATPase) that pumps protons across the plasma membrane, creating a higher concentration of H+ in the lumen, compared with the cytoplasm. A cation-proton antiporter uses the higher H+ concentration in the lumen to pump K+ and Na+ against a concentration gradient. Thus, the cation-proton antiporter is a secondary active transporter that can move K+ and Na+ “uphill” using the energy supplied by the H+ gradient that the V-ATPase creates. The accumulation of cations in the lumen creates a voltage that makes the luminal side side of the tubule wall positively charged compared with the hemolymph side, attracting negatively charged Cl– ions from the hemolymph. Most of the Cl– ions move through a calcium-dependent chloride channel (Ca-ClC). The increase in solutes attracts water by osmosis from the hemolymph, through the water channel aquaporin (described below). Thus, Malpighian tubules absorb solutes such as K+, Na+, Cl–, and nitrogenous wastes from the hemolymph, and water follows the solute by osmosis. The joint movement of salts and water results in a filtrate that is isosmotic with the hemolymph. Ions, water, and nitrogenous waste products move down the hindgut of the digestive tract, but near its end, ions and water are reabsorbed into the hemolymph, while waste products are excreted into the feces. Despite the lack of mechanical filtration, Malpighian tubules represent an effective way to get rid of nitrogenous wastes while properly maintaining an internal ionic balance.
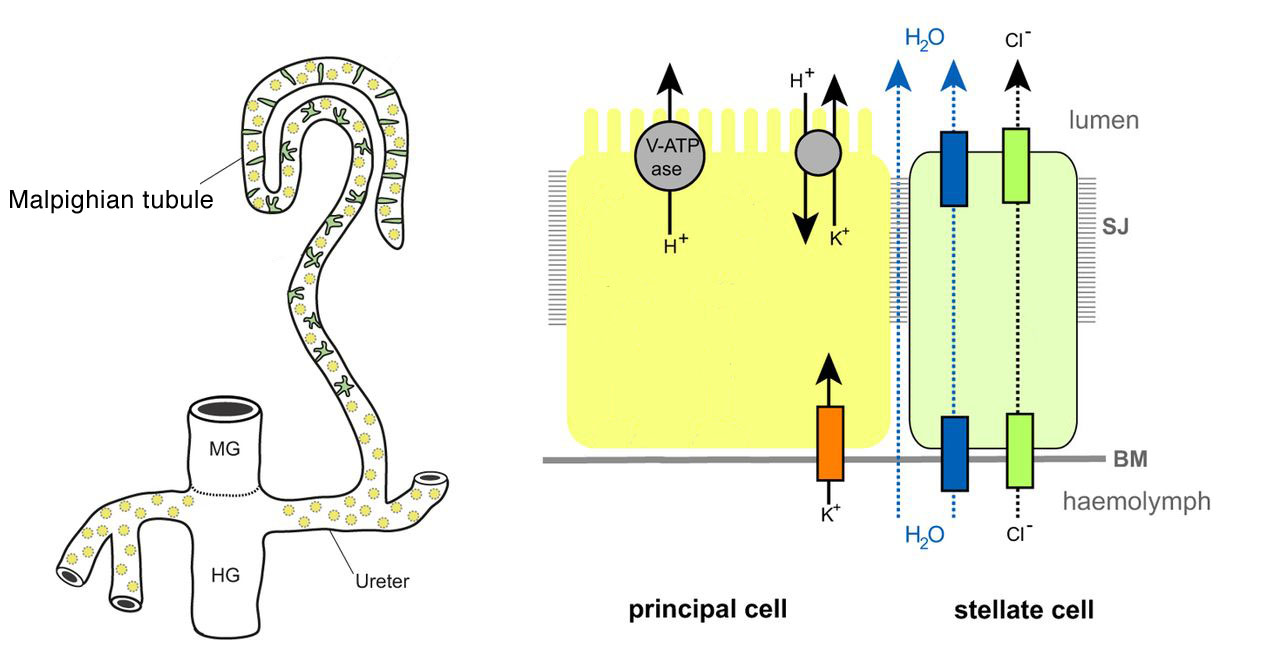
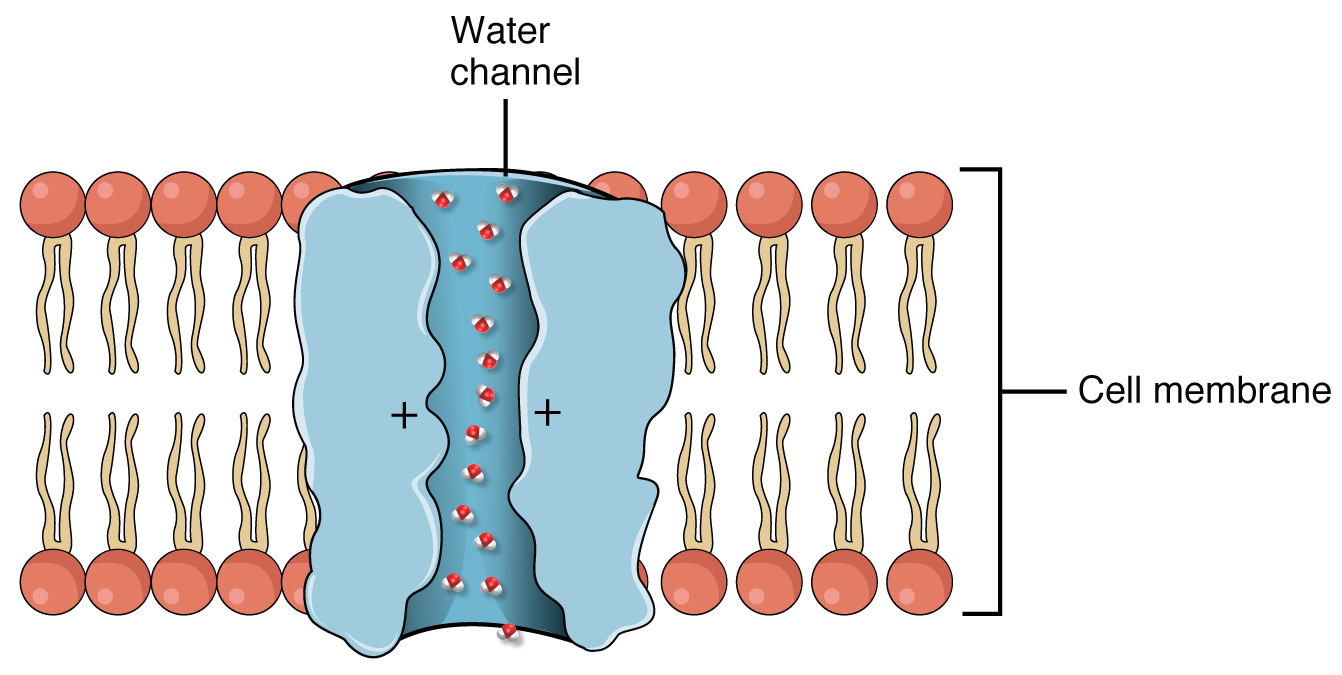

9.7 The Kidneys
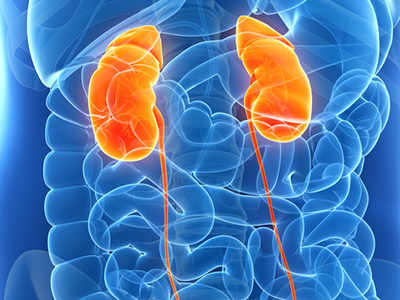
So far in this chapter, each renal organ we’ve looked at functions as a single tubule. In larger, more complicated animals, there’s often an advantage to grouping thousands or even millions of tubules together into a single organ. An organ encapsulating many renal tubules is termed a kidney.
The kidney is a complex organ that performs multiple functions simultaneously. The primary function is to regulate the composition of the extracellular fluid by selectively adjusting the composition of the plasma that circulates through its blood vessels. Materials to be conserved are retained in the plasma, while waste products are extracted and excreted.
The functions of the kidney include:
- Filtering the blood;
- Regulation of water and ion balance by controlling the volume of various body fluid compartments, osmolality, acid-base balance, and concentration of several electrolytes;
- Removal of toxins and metabolic waste products, particularly excess nitrogen from the breakdown of proteins and nucleic acids, while keeping essential blood components that body needs to function normally;
- Production of hormones, and control of blood pressure.
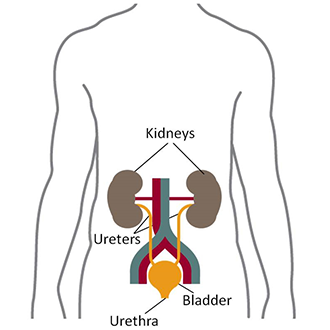
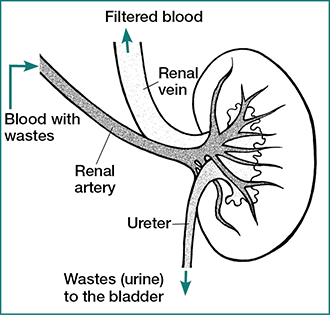
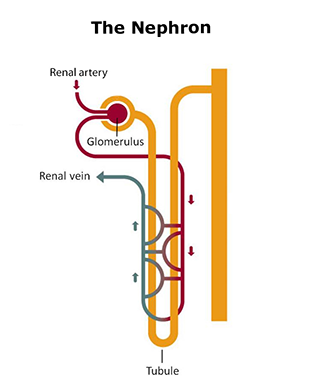
9.7.1 Evolutionary Adaptations of the Kidney
The structure of the kidney varies across groups of vertebrates, due to evolutionary adaptation to different environmental conditions. As a consequence, in some species, kidneys function mostly to conserve water, while in other species, the kidneys function mostly to remove water from the body. A freshwater fish, for example, does not drink water, but produces copious dilute urine, while a saltwater fish drinks large amounts of water, and needs to get rid of the excess of salts by producing concentrated urine. Thus, animals living in extreme conditions and those with different diets are associated with substantial differences in renal structure and therefore in functional specialization:
Freshwater fish living in non-salty environments have kidneys adapted for rapid filtering of the blood, retaining salts and producing very dilute urine.
Many amphibians have permeable skin that absorb fresh water from their moist environment, consequently have kidneys similar to freshwater fish.
Marine fish, and desert mammals living in environment with little or no access to water, have kidneys adapted to conserve water therefore they produce concentrated urine.
Carnivores and insectivores consume high-protein meals, therefore their kidneys are adapted to dispose of large amounts of nitrogenous waste.
Hematophagous animals – animals that feed primarily on blood of other animals – incorporate large amounts of water and salt into their system, consequently their kidneys are adapted to excrete a large amount of urine while retaining nutrients. This is on top of the challenge of getting rid of the nitrogenous waste from a diet that is mostly protein. For example, the vampire bat (Desmodus rotundus) drinks half its body weight or more in a single meal, so much blood that the bat has difficulty flying before producing a lot of urine. The urine has ten times the osmotic pressure of the bat’s own tissues, mostly from concentrated urea. Here in Canada, our abundance of sea lampreys makes up for our lack of vampire bats and candiru fish5.
Hibernators may spend several months without feeding, neither defecating nor urinating during this time. Examples of hibernators include bears, skunks, groundhogs, and bats. During hibernation, black bears and brown bears rely on their body stores of fat and protein for energy. Because they don’t urinate, urea from protein catabolism can’t be eliminated. Then why doesn’t urea accumulate during hibernation? Surprisingly, blood urea actually drops instead of rising during hibernation due to the unusual ability of the bear to recycle nitrogenous waste to make new protein.

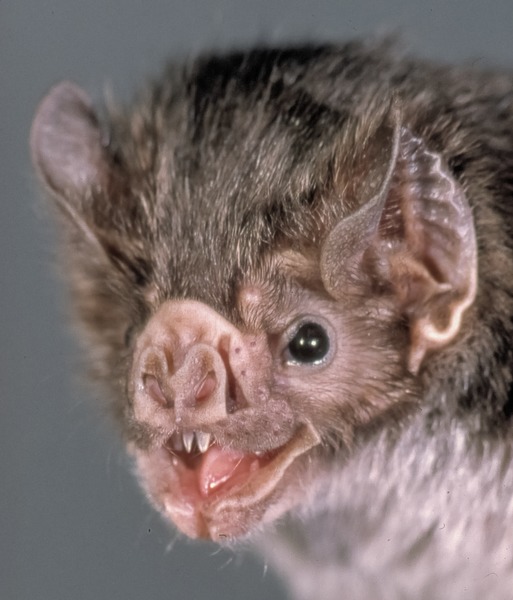
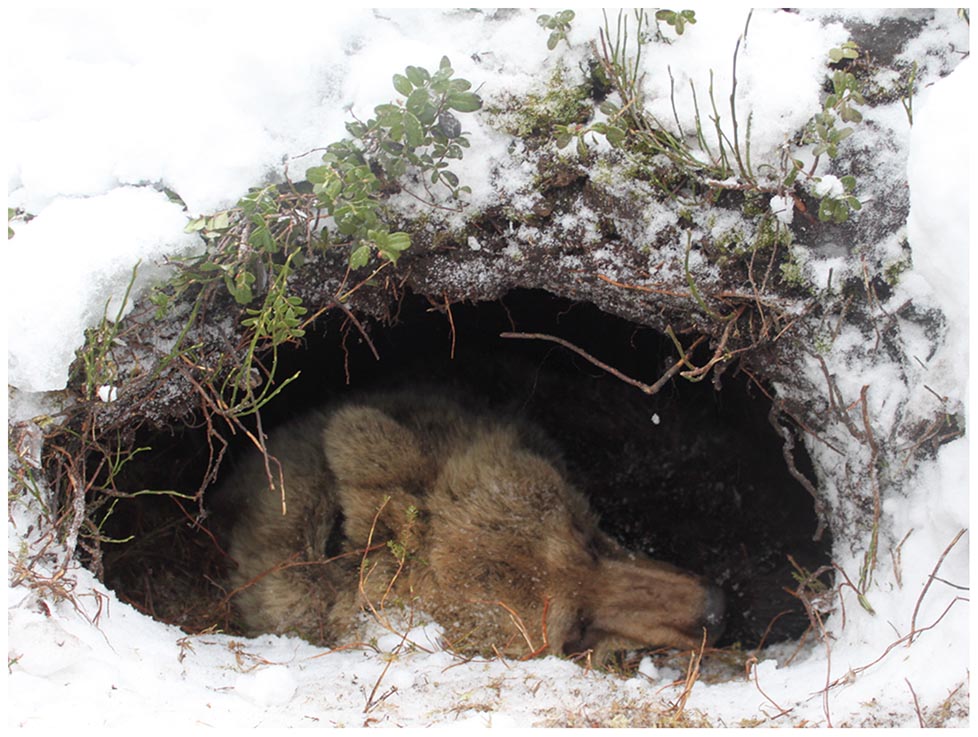
Group of Animals | Blood Concentration Relative to Environment | Urine Concentration Relative to Environment | Main Nitrogen Waste | Osmoregulatory Mechanism & Organ |
Marine Fishes | Hypoosmotic | Isoosmotic | Ammonia | Drink seawater, secrete salt from gills |
Freshwater Fishes | Hyperosmotic | Strongly hypoosmotic | Ammonia | Drinks no water, transport salt across the gills |
Freshwater Amphibians | Hyperosmotic | Strongly hypoosmotic | Most secrete urea | Absorbs water through skin |
Marine Reptiles | Hypoosmotic | Isoosmotic | Ammonia, urea, uric acid | Drinks seawater, hyperosmotc salt gland secretion |
Terrestrial Reptiles | Isoosmotic | Uric Acid | Drinks fresh water | |
Marine Birds | Hypoosmotic | Weakly hyperosmotic | Uric Acid | Drinks seawater, hyperosmotic salt gland secretion |
Terrestrial Birds | Weakly hyperosmotic | Uric Acid | Drinks fresh water | |
Desert Mammals | Strongly hyperosmotic | Urea | Drinks no water; highly specialized kidney with very long loops of Henle; depends entirely on water generated by cellular metabolism | |
Marine Mammals | Hypoosmotic | Strongly hyperosmotic | Urea | Drinks no water |
Other mammals | Strongly hyperosmotic | Urea | Drinks fresh water | |

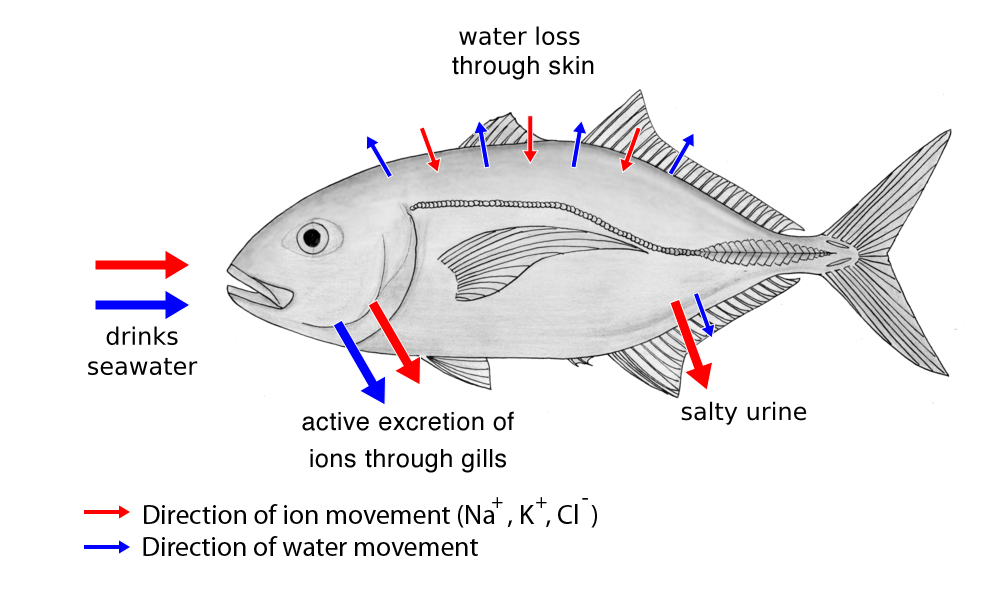
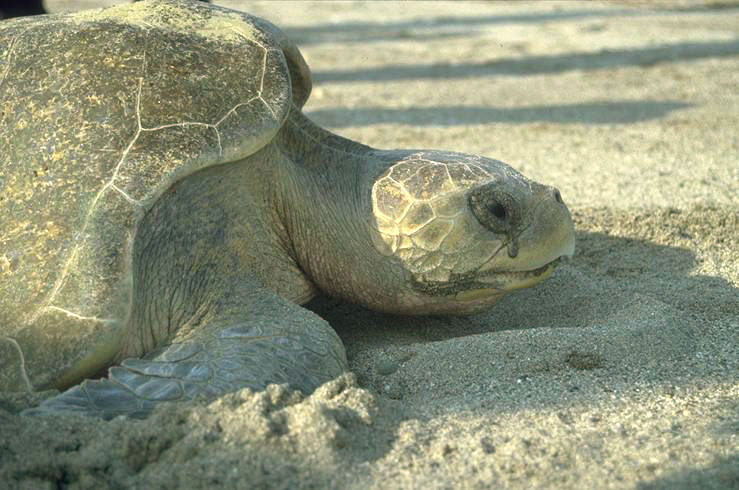

9.7.2 Relationship of Kidney Structure to Function
Kidneys are paired organs located on the abdominal cavity on each side of the aorta. Mammalian kidneys are bean-shaped with a smooth convex border and a recessed concave border termed the hilum, where the renal artery enters and the renal vein and the ureter carrying the urine leaves the organ.
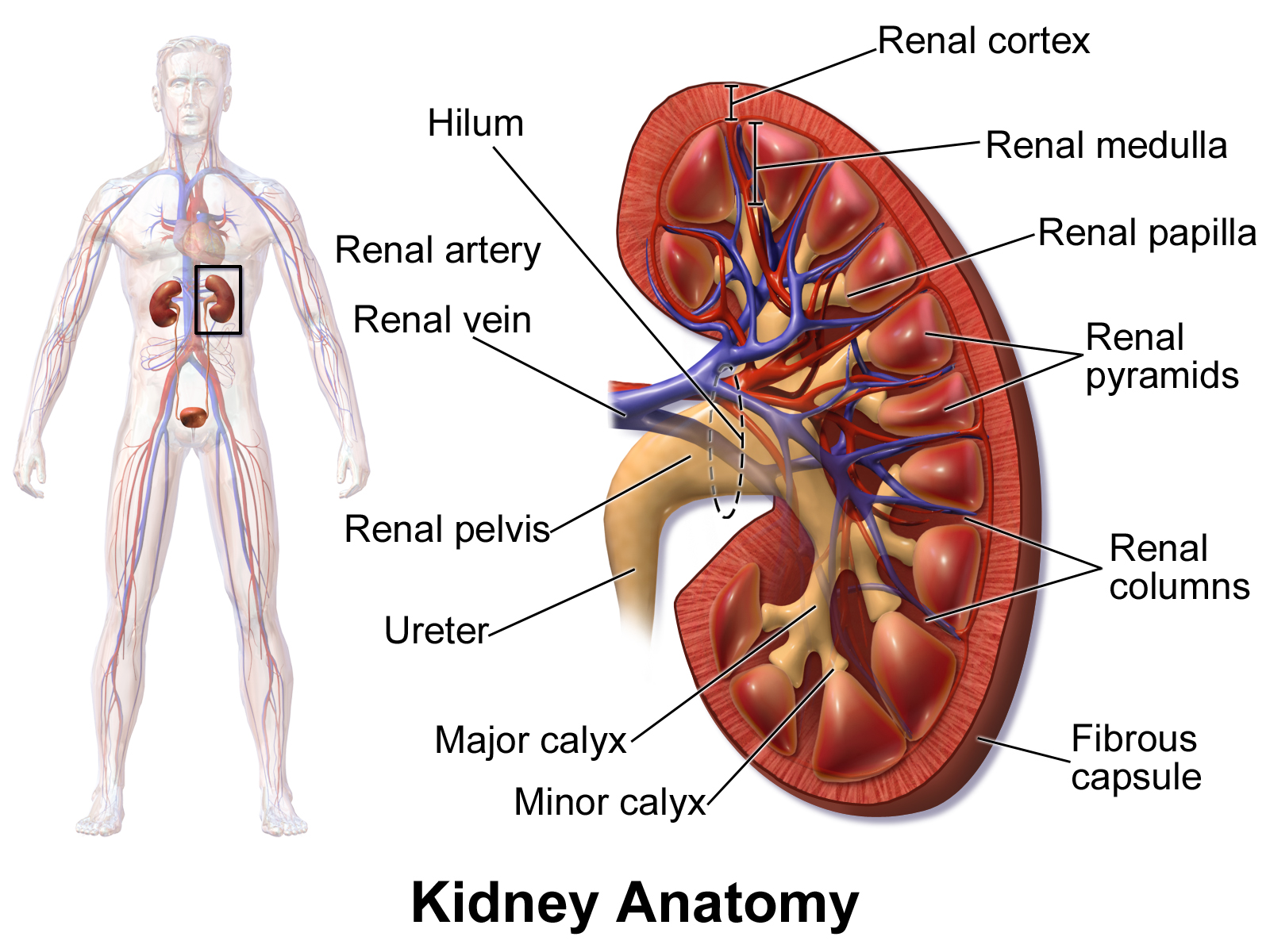
The concept that “form follows function”6 used by architects can be applied to relate shape to function, a principle that also applies to organs, cells, organelles, and biological molecules, though we could also say “form defines function.” Thus, by carefully examining the structure of the kidney, we can predict some of its function; and by associating the diet and habitat of different animal species with the structure of their kidneys, we can speculate on how evolutionary environmental pressures modified the architecture of the organ to adapt to their osmoregulatory and waste disposal needs.
Two visible anatomical regions can be distinguished in the kidney:
- an outer cortex (Latin: bark of a tree) and
- an inner medulla (Latin: bone marrow, from Latin, medius: middle).
The cortex has a granular (grainy) appearance, while the medulla has striations (stripes) that are finer in the inner part of the organ than in the outer medullary region. The kidney is divided into lobes or pyramids, which are the macroscopic functional unit7 of the organ, while the microscopic functional unit is the nephron. The nephron has two well-defined parts:
- the renal corpuscle containing a cluster of capillaries called the glomerulus, and
- a duct called the renal tubule.

Two populations of nephrons can be distinguished in the kidney:
- the cortical nephrons located near the surface of the kidney, and
- the juxtamedullary nephrons located close to the border between cortex and medulla.
Cortical nephrons are much more abundant in the kidney than juxtamedullary nephrons, but the juxtamedullary nephrons are important for creating and maintaining an osmotic gradient across the renal medulla that’s needed to concentrate the urine.

The renal corpuscle consists of a cluster of capillaries, known as glomerulus, which is the structure that performs the first step in the formation of urine. The glomerulus is surrounded by a thin-walled, saclike structure of connective tissue termed the Bowman’s capsule, which is surrounded by a thin sheet of extracellular matrix protein and is comprised of three layers in the interior:
- Parietal layer – a single layer of squamous epithelium, forming the wall of the capsule to contain the primary filtrate;
- The capsular space – the space (lumen) where the primary filtrate collects; and
- The visceral layer – contains cells called podocytes (Greek, podo: foot), with long projections, called foot processes, or pedicels8, that wrap around the capillaries and leave filtration slits between them forming the filtration barrier, which is a sort of diaphragm located between the feet or processes and works as a molecular sieve. Blood filters through the filtration slit fluid leaving the glomerular capillaries to enter in the lumen of the Bowman’s capsule.
The glomerulus is a physical filter, and glomerular filtration into the surrounding Bowman’s capsule is the first step in the process of producing urine. Blood pressure through the capillaries is the driving force for glomerular filtration. The filtration barrier of the glomerulus is highly permeable and nearly 20% of the plasma volume entering the glomerular capillaries is filtered. Water and small solutes present in the blood, interstitial fluid pass through the glomerulus leaving in the general circulation the cellular components of blood, large solutes and negatively charged proteins. The fluid that passes through the glomerulus and into the capsular space enters a highly convoluted tubular structure termed into the proximal convoluted tubule (PCT; proximal means adjacent to with respect to the nephron).
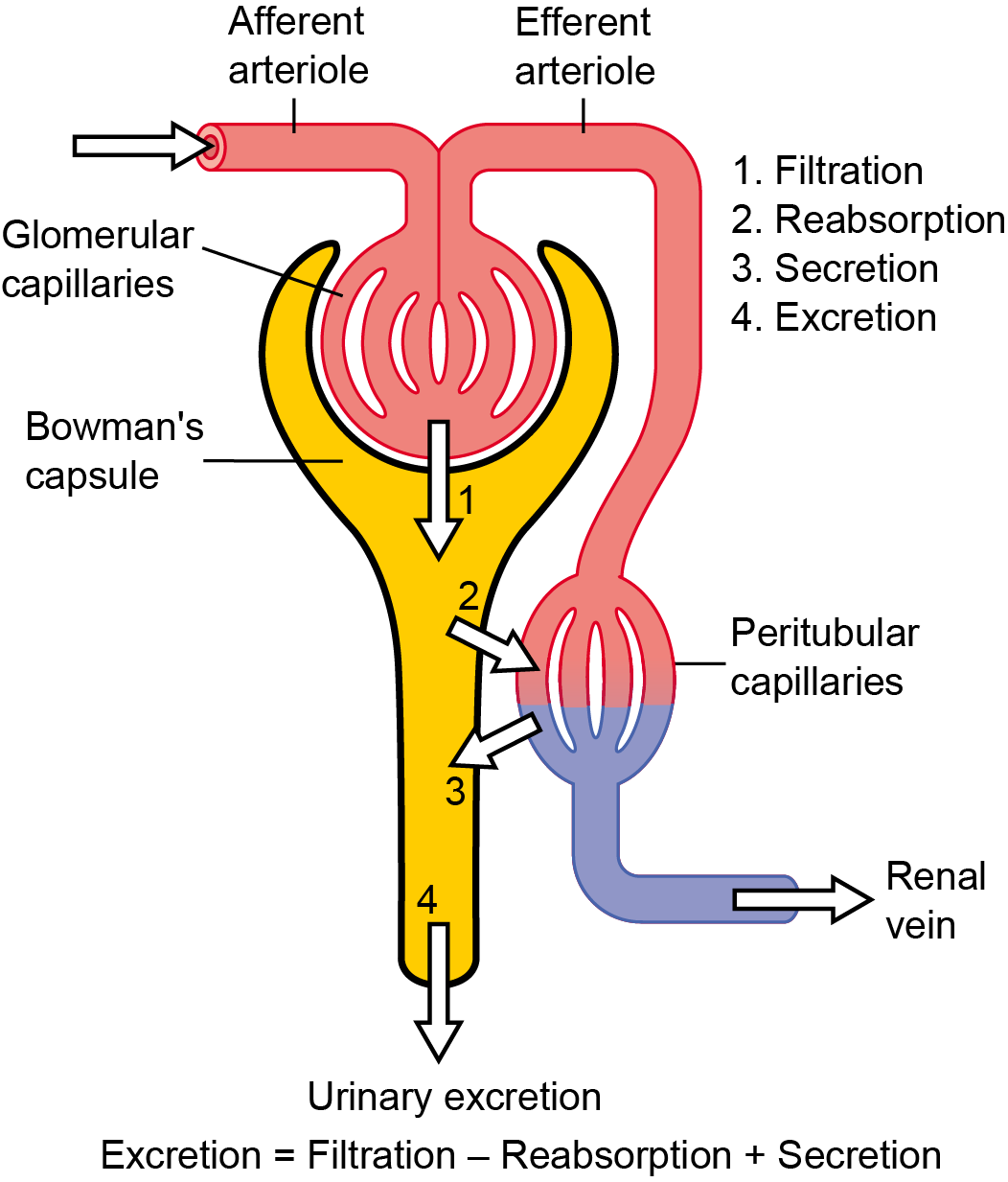
The Bowman’s capsule has two poles, the vascular pole and the urinary pole. The vascular pole has an anatomical particularity: the blood entering through an afferent arteriole also exits through an arteriole, the efferent arteriole, instead of a venule as in other organs. This anatomical feature has a functional role since constriction of the efferent arterioles as blood exits the glomerulus provides resistance to blood flow, preventing a pressure drop, which would not be achieved if blood were to flow into venules, because they do not constrict. The urinary pole is the side of the Bowman’s capsule where the tubule of the nephron begins.
Each human kidney has about 1 million nephrons of which two basic subtypes can be distinguished depending on the length of the loop of Henle. The juxtamedullary nephrons have long loops of Henle that dip far into the renal medulla. A group of straight capillaries termed vasa recta (Latin, vasa: vessels; recta: straight; described below) lie in parallel to the loop of Henle. The cortical nephrons have short loops of Henle that do not dip far into the renal medulla and do not contain vasa recta.


Legend:
A Renal corpuscle
B Proximal tubule
C Distal convoluted tubule
D Juxtaglomerular apparatus
1 Basement membrane (basal lamina)
2 Bowman’s capsule – parietal layer
3 Bowman’s capsule – visceral layer
3a Pedicels (foot processes of podocytes)
3b Podocyte
4 Bowman’s space (urinary space)
5a Mesangium – Intraglomerular cell
5b Mesangium – Extraglomerular cell
6 Granular cells (juxtaglomerular cells)
7 Macula densa
8 Myocytes (cells of smooth muscle)
9 Afferent arteriole
10 Glomerulus capillaries
11 Efferent arteriole
9.7.3 The Kidney’s Tubules
The fluid filtered by the glomerulus flows into a straight tubular section towards the medulla, termed the thick limb of the Henle’s loop (HL). After penetrating the medulla, the tubule turns back and re-enters the cortex, forming a hairpin loop. Thus, according to its anatomical position the HL is divided into a descending limb (descending into the medulla) and an ascending limb (ascending back into the cortex).
The ascending limb of the HL connects with the distal convoluted tubule (DCT) located farthest from the glomerulus, which extends forming the connecting tubule (CNT, or junctional tubule). Finally, urine flows through the collecting ducts (CD) into the renal pelvis and is disposed through the ureter into the urinary bladder, where the urine collects.
Aquaporins (AQP) are integral membrane proteins that serve as channels in the transfer of water, and in some cases, small solutes across the membrane. Structural analyses of the molecules have revealed the presence of a pore in the center of each (AQP) molecule. More than 10 isoforms (AQP0-AQP10) have been identified in mammals. They are differentially expressed in many types of cells and tissues in the body. AQP1 is found in the blood vessels, kidney proximal convoluted tubules. AQP2 is expressed in the kidney collecting ducts, where it shuttles between the intracellular storage sites and the plasma membrane under the control of antidiuretic hormone (ADH). AQP3 and AQP4 are present in the kidney and urinary tract. AQP5 is localized in intracellular vesicles of the kidney collecting duct cells, while AQP8 is widely distributed kidney. AQP8 is expressed in the kidney, testis, and liver. AQP9 is present in the liver and leukocytes.
The cell composition, configuration and length of the tubules vary with the location of the nephron within the kidney and also between species, supporting the concept that form defines function. The lumen of the nephron is entirely lined by epithelial cells, but the cell types along the tubule are different according to their specific function.
Cells in the PCT have large concentrations of mitochondria located near the basal surface, marked basal membrane infoldings, and a well-developed brush border facing the lumen of the tubule. The large number of mitochondria suggests high metabolic activity of cells that are involved in reabsorption of glucose and amino acids; while the brush border, relaxed cell junctions and rich in aquaporins (AQPs; described in the textbox) suggest an epithelium highly permeable to solutes and water.
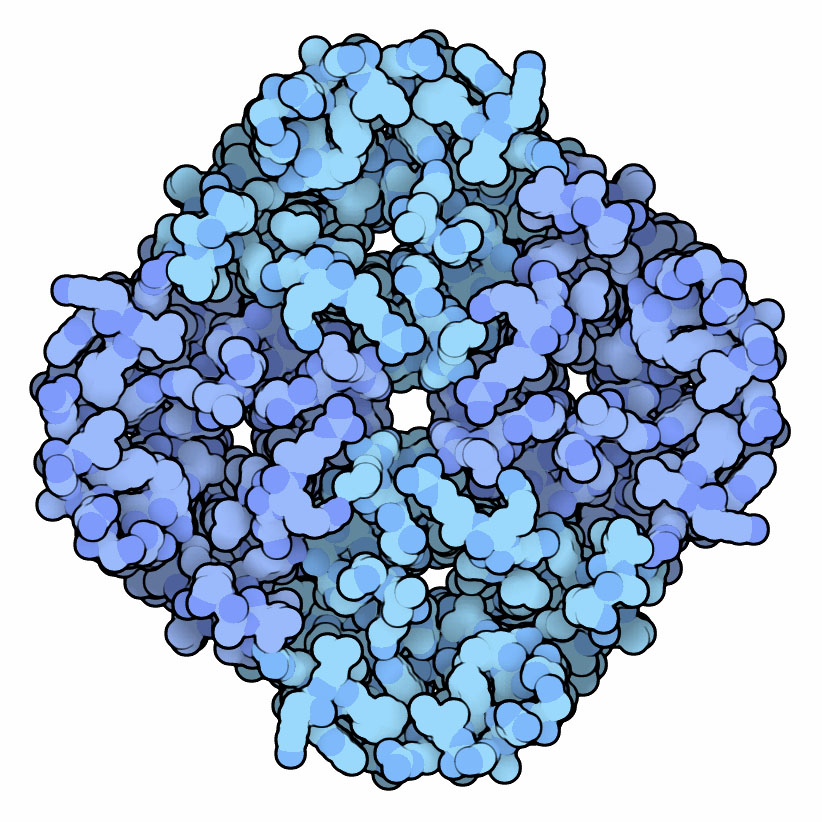
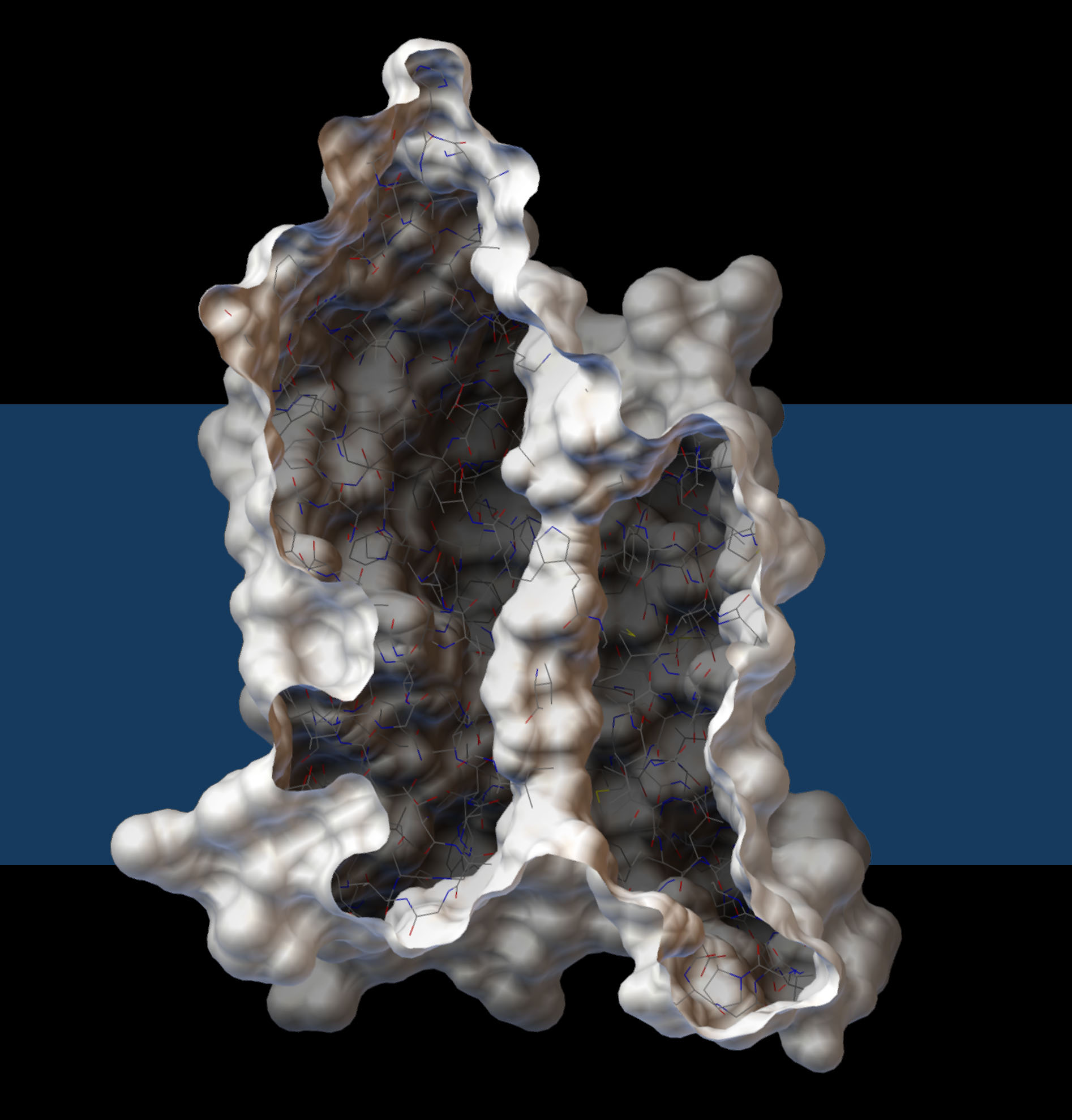
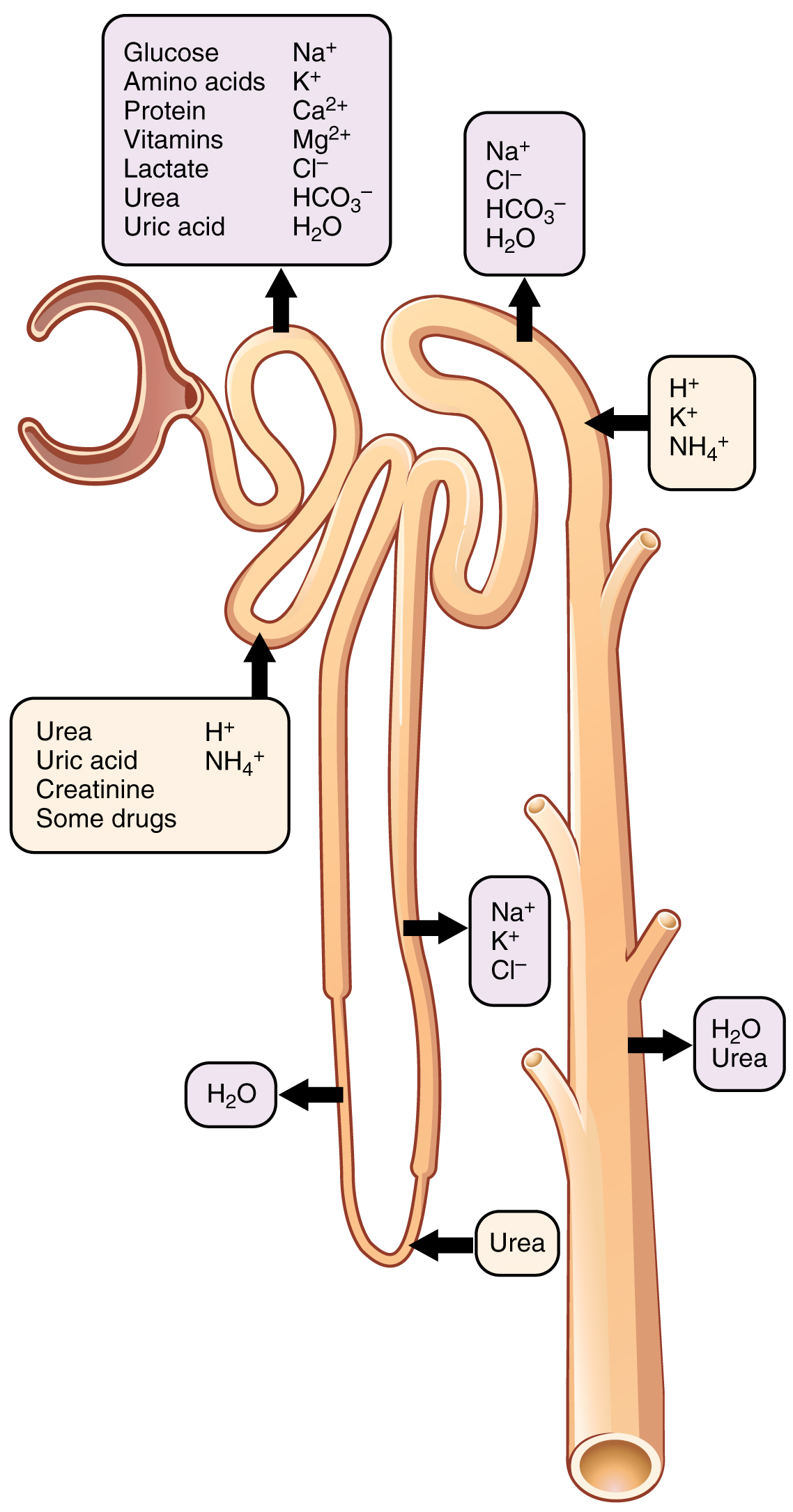
Between two-thirds and 100% of solute filtered into the Bowman’s capsule is reabsorbed in the PCT, including Na+, K+, Cl–, HCO3–, Ca2+, and organic molecules, such as glucose and amino acids. Some ions diffuse through membrane ion channels into the epithelial cells of the PCT, while other solutes are actively transported across the tubule. Organic molecules generally are reabsorbed by transporters, such as the Na+/glucose symporter, which is a secondary active transport mechanism driven by the Na+/K+ ATPase located on the basolateral membrane of the PCT cells. The Na+/K+ ATPase moves 3 Na+ outward into the ECF while bringing 2 K+ into the cell, generating a downhill Na+ gradient from the lumen of the tubule into the epithelial cell of PCT. Glucose is in high concentration inside the epithelial cell therefore needs to be transported down its concentration gradient. The energy generated by the Na+/K+ ATPase is used by the sodium-glucose transporter (SGLT), located on the lumen side of the PCT cell membrane, to transport glucose ‘uphill’, up the glucose gradient into the cell and into the peritubular capillaries.
Cotransport is the process by which two substances are simultaneously transported across a membrane by a protein complex without ATPase activity. Cotransporters bind to two molecules or ions at a time and use the gradient of one of the solutes to force the other solute against its concentration gradient. The energy used by cotransporters derives from the chemical energy generated by ATP dependent primary active transporters.
Thus, tubular reabsorption9 is the process by which the nephron recaptures valuable solutes that passed through the glomerulus by filtration. The sodium-glucose transport is a mechanism of facilitated diffusion that uses a specific solute binding protein as a carrier (Figure 19.4 Stanfield). When all the binding sites for the solute are occupied, the system is operating at what is called transport maximum (Tm). In the case of glucose the Tm is very much above the normal concentration of the sugar in blood, thus in normal conditions glucose is completely reabsorbed in the PCT. However, when blood content is above the Tm the glucose carrier saturates and glucose is loss in the urine.
In humans, the Tm for glucose reabsorption is 375 mg/min. At a normal plasma glucose concentration of 80-100 mg/dL, and a glomerular filtration rate (GFR) of 125 mL of plasma per minute the filtered load of glucose is between 100 and 125 mg/min, which is very much below the glucose Tm. Thus at normal blood concentration, glucose is completely reabsorbed in the PCT, so it is not present in the final urine of a normal individual.
Cells of the straight portions of the PCT and thick limbs of HL have fewer mitochondria and sparser brush borders, which are an indication of water permeability but less active transport of solutes, therefore low ions permeability. Thus, in the descending HL water is reabsorbed by the high osmotic pressure of the peritubular interstitium exerted by the solutes reabsorbed in the PCT.
Cells of the ascending limb of the Henle’s loop have a much lower number of aquaporin channels, therefore they are impermeable to water. However, they are highly permeable to ions that create an even stronger osmotic gradient in the interstitium. The osmotic gradient is higher as it approaches the limb region of the medulla. Overall the dual permeability to water and the continuous flow of ions to the interstitium causes an outflow of water from the lumen of the tubule to the ECF.
The DCT is composed of two main groups of cells, the principal cells, and the intercalated cells, also termed dark cells. Principal cells are intermediate between the cells of the PCT and those of the descending HL. On the lumen side, principal cells have a Na+-Cl– cotransporter. They are involved in reabsorbing Na+ and Cl–, and secreting K+. Reabsorption of Na+ by the principal cells of the DCT is regulated by the steroid hormone aldosterone, secreted by the cortex of the adrenal glands.
Principal cells also have a Ca2+ channel in the lumen side of the DCT, which is controlled by parathormone (PTH), a peptide hormone secreted by the parathyroid glands. Although Ca2+ is reabsorbed throughout the tubule, mostly in the PCT, PTH regulates reabsorption Ca2+ in the DCT.
Intercalated cells of the DCT have prominent microvilli, are rounder than their neighbours and play a major role in the secretion of protons and absorbing bicarbonates, thus are involved in regulating urine pH.
Cells of the collecting duct form a cuboidal epithelium that plays a major role in the hormonally regulated excretion of water. These cells express the water channel AQP2 that responds to the hormone vasopressin, also called antidiuretic hormone (ADH). The hypothalamus produces vasopressin, but it’s secreted by the posterior pituitary gland. Vasopressin increases water permeability of the collecting duct by inducing translocation of AQP2 the plasma membrane facing the lumen side of the collecting duct.
Overall, the architecture and cell composition of the tubules follows the principle of “form follows function.” An abundance of mitochondria and a thick brush border on the lumen side of the epithelium are associated with transport of large quantities of salt and water, while regions of the tubule with fewer mitochondria and sparser brush border are associated with low transport of salts and impermeability to water.
The length of tubules varies with the site of origin of the nephron. Henle’s loops of cortical nephrons are short and thick over much of their length. In contrast, juxtamedullary nephrons originate deeper within the cortex and are much longer. The descending limb of the typical justamedullary nephron is very thin and penetrate the entire length of the medulla. The length of the Loop of Henle defines the ability to concentrate urine in different animal species. Shorter renal tubules are found in animals living in environments with abundant water at their disposal, while very long tubules are found in species living in dry environments.
The renal pelvis is an enlarged tubular compartment shaped like a funnel with cuplike extensions, called calyces. It is formed by tubules emerging from the renal medulla with conical shaped subdivisions termed renal pyramids. The apex of the renal pyramids, termed renal papilla, face the calyces. Minor calyces converge and form a major calyx communicating the renal pelvis with the ureter, which receive urine that flow from the kidney to the urinary bladder or a cloaca.
9.7.4 Circulation in the Kidney
In addition to providing oxygen and nutrients, blood enters the kidney primarily to be cleansed. The kidney regulates the composition of the body’s extracellular fluid by selectively adjusting the composition of the plasma that flows through the renal vasculature.
Thus, despite their relatively small size, the kidneys receive approximately 20% of the cardiac output of blood, and produce one ml of urine per 1.3 liters of blood that circulate into the organ. The human kidney filter approximately 3 liters of plasma every 22 minutes. Accordingly, the kidney is a highly vascularized organ, receiving blood from the abdominal aorta, which branch into the renal arteries and further divides into several segments that reach the renal lobes and runs up between the pyramids. Circulation in the kidney is unique in several aspects:
- A high volume of blood flow in the kidney through a countercurrent system10 (described below), where arterial blood is taken into close proximity to the venous blood flowing in the opposite direction;
- This countercurrent system creates large functional arteriovenous shunts11 that allows blood to flow from an artery to a vein without going through a capillary network;
- The renal circulation has two capillary circulations in tandem; both the pre- and post-capillary blood vessels in the glomerulus are arterioles;
- An afferent arteriole irrigates the glomerulus, while an efferent arteriole move into the peritubular capillaries, which are tiny capillaries that surround the PCT, the descending and ascending parts of the loop of Henle and DCT.
- The efferent arteriole of the renal corpuscle is structurally and functionally an artery and not a venule, since it ramifies into the peritubular capillaries;
- The peritubular capillaries form an efferent venule, which combines venules from other nephrons and join the venous stream.
- From the efferent arterioles vessels branch of forming the vasa recta, which is a straight arteriole that enter the medulla. Each vasa recta has an hairpin turn surrounding the loop of Henle. This anatomical distribution of the vasa recta makes blood to circulate at a very slow rate in the medulla, preventing the washout of the osmotic gradients generated in the medulla by countercurrent exchange (described below).

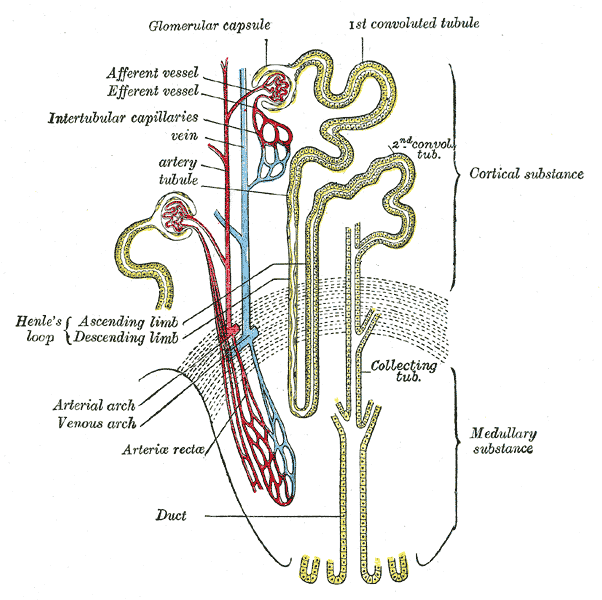
9.7.5 Osmotic Gradient in the Kidney
The goal of the entire process of urine formation is to restore valuable solutes to the internal media conducting to the production of a hyposmotic fluid with the disposable wastes. The ability of the kidney to produce hyposmotic urine depends upon the generation of a hyperosmotic environment in the interstitial fluid surrounding and along the loop of Henle. This hyperosmotic environment is not uniform along the kidney; it is continuously generated in a form of an increasing gradient of osmolarities between the cortex and the medulla. Depending on the species the osmolality varies from 300 mOsm/kg at the border of the medulla to approximately 1200-1400 mOsm/kg at deepest part of the medulla, near the renal pelvis.
The loops of Henle create this gradient by permitting diffusion of water and salts in the descending limb, while limiting movement of water and reabsorbing solutes in the ascending limb. The mechanism of generating this gradient is termed a countercurrent multiplier, because by circulating fluids through the loop of Henle in opposite directions the kidney is able to multiply its capacity of solute reabsorption from the collecting ducts into the interstitial fluid.
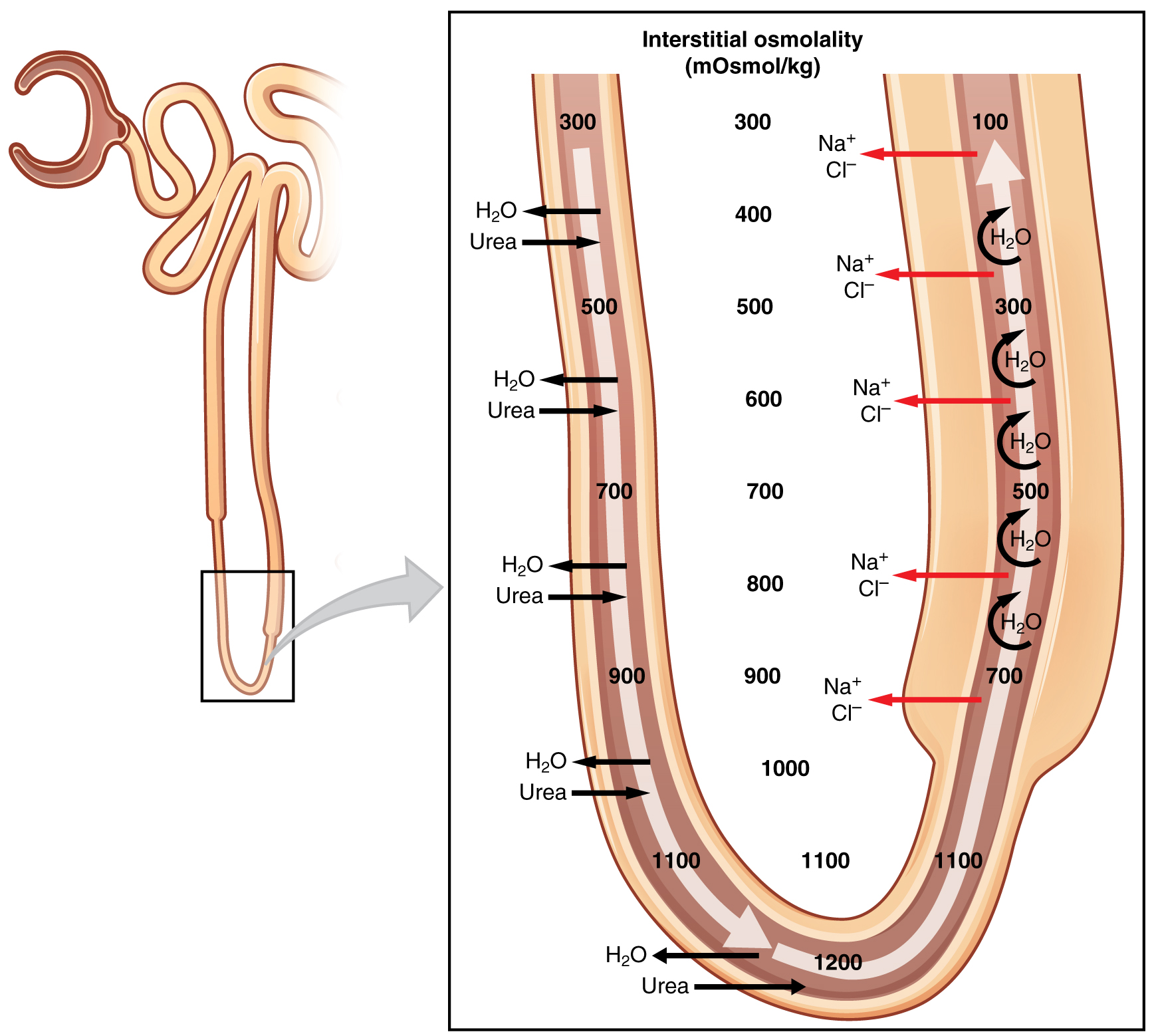
9.7.6 The Countercurrent Multiplier System
The term countercurrent derives from the structure/function relationship of the loop of Henle, where the fluid that flow through the descending limb move in parallel to the ascending limb but in opposite directions. Countercurrent is very dynamic mechanism that in real time it occurs simultaneously with the rest of the functions of the kidney. However, for the purpose of description must be divided into different stages.
- When the filtrate moves from the PCT into the descending limb of the loop of Henle the osmotic pressures between the tube and the interstitial fluid, are the same – around 300 mOsm/kg – because water moves freely and is re-absorbed along with solutes. Therefore, as the fluid moves down the descending limb, there is no net gain of water in the peritubular fluid.
- But as the fluid move up the ascending limb of the loop of Henle, Na+, Cl–, and K+ are actively transported from the tubule into the interstitial fluid, increasing the osmolality of the medullary peritubular interstitial fluid from the original 300 mOsm/kg to 400 mOsm/kg, while lowering the osmolality of the tubular fluid to 200 mOsm/kg.
- Because this increase in osmolarity in the interstitial fluid affect both limbs of the loop of Henle, the fluid in the descending limb that is at 200 mOsm/kg move to the peritubular fluids, which is now at 400 mOsm/kg.
- As the nephron continue filtrating blood, more fluid at 300 mOsm/kg enter from the PCT into the loop of Henle pushing the fluid with higher osmolarity deeper into the descending limb.
- Thus, the fluid at 400 mOsm/kg moves up into the ascending limb where active transport of Na+, Cl–, and K+ raises the osmolarity of deeper medullary interstitial fluid from 400 mOsm/kg to 500 mOsm/kg.
- This increase in interstitial fluid osmolarity moves more water from the descending limb into the medullary interstitial fluid.
- Now the descending limb is iso-osmotic with the medullary interstitial fluid, but more filtrate at 300 mOsm/kg continue entering from the PCT, pushing the higher osmolality fluid toward the tip of the loop of Henle.
- The process continues until the medullary osmotic gradient is created and the system reaches a steady state.
- At steady state, the fluid entering the loop of Henle from the PCT is always iso-osmotic to interstitial fluid, at 300 mOsm/kg, but the osmolality of the tubular fluid within both limbs can reach up to 1400 mOsm/kg at the tip of the loop of Henle deeper in the medulla.
At any given position in the osmotic gradient of the medulla, the osmolality of the fluid in the ascending limb is always lower than the osmolality of fluid in the descending limb because the ascending limb is impermeable to water and actively transports solutes out of the tubular fluid. As the tubular fluid leaves the loop of Henle and enters the distal tubule, it is hypo-osmotic to extracellular fluid at approximately 100-200 mOsm/kg. At the end of the process, the countercurrent system retains solutes and generates a hyposmotic urine.

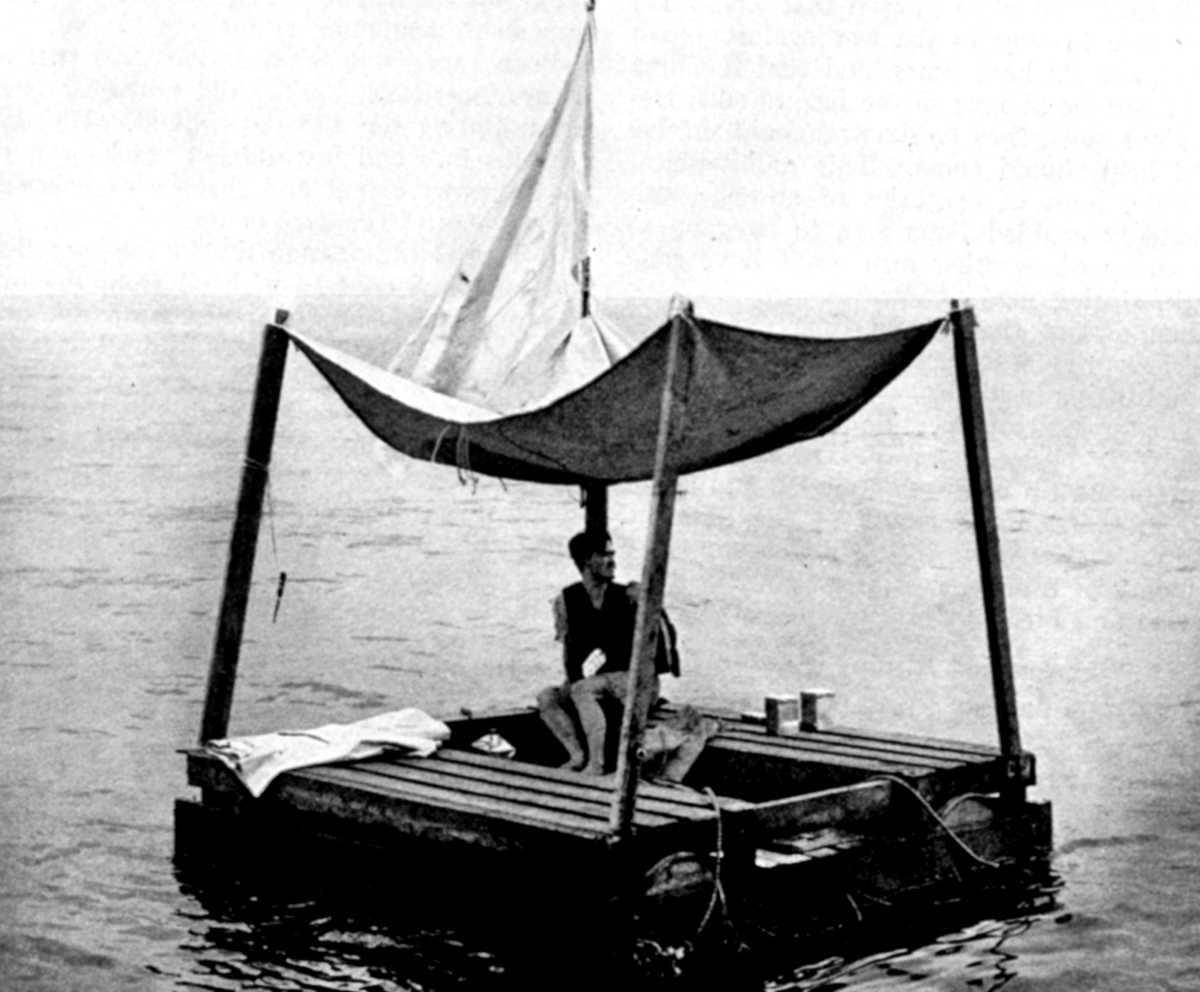
Photo: US Navy, public domain, 1945.
Water, water, everywhere,
And all the boards did shrink12;
Water, water, everywhere,
Nor any drop to drink.
– Samuel Taylor Coleridge, The Rime of the Ancient Mariner (1798; 1817)
Why can’t you drink seawater to stay hydrated? For thousands of years, it’s been a frustration for sailors adrift at sea. They’re surrounded by water, but they can’t drink it. The reason is that seawater is around 1050 mOsm/kg, while the medulla at the tip of the human renal papilla is, at most, 1200-1400 mOsm/kg. The final step of concentrating urine is osmosis of water from the collecting ducts into the surrounding medulla, so the maximum salt concentration in human urine is only a little bit higher than seawater. So most of the water ingested would have to be used to get rid of the extra salt. Also, the human digestive system would have difficulty absorbing much fluid from seawater, since human blood is only 300 mOsm/kg. Most likely, attempting to drink enough seawater to stay hydrated would lead to diarrhea that would dehydrate the body even further.
How did Poon Lim manage to survive so long on a raft on the ocean? His supplies of water and food ran out after one month at sea. He did collect and drink rainwater, but that probably wasn’t quite enough water to stay alive for the remaining three months. So, how did he do it? The answer is hidden in Table 9.1 of this chapter13.
9.7.7 Endocrine Role of the Kidney
In addition to being a blood filter, the kidney is an important endocrine gland. Thus, the kidney has two independent functions:
- urine production, and
- hormone production.
Hormones made by the kidney include:
- erythropoietin,
- calcitriol (1,25 dihydroxyvitamin D), and
- renin.
Erythropoietin
How does the body control the quantity of red blood cells in the blood? Too few erythrocytes, and the blood wouldn’t be able to deliver enough oxygen to tissues. Too many, and the blood would be too viscous to maintain enough blood flow.
In humans and other mammals, the kidney is the organ that measures the oxygen supply to adjust the amount of red blood cells in the blood. If the amount of oxygen supplied by the blood drops, the slight shortage of oxygen triggers increased production of erythropoietin, a chemical signal that tells bone marrow cells to multiply and mature into red blood cells. When the amount of red blood cells rises, the increased oxygen carrying capacity of the blood increases the oxygen supply to the kidney, reducing the production of erythropoietin, resulting in reduced red blood cell production by the bone marrow. Thus, erythropoietin acts as a circulating hormone to carry a message from the kidneys to the bone marrow. Because destruction of erythropoietin is constant, with a half-life of five hours in blood, erythropoietin levels closely reflect the long-term oxygen supply.
You might have heard of erythropoietin before, or EPO for short – it’s relatively easy to make large amounts of artificial erythropoietin, because erythropoietin is a protein that can be expressed in cell culture using recombinant DNA technology. In competitive sports, many athletes have had themselves injected with artificial erythropoietin to boost their athletic performance by boosting the oxygen carrying capacity of the blood. Because EPO is so frequently abused by athletes, EPO doping is banned in most official sports.
The main source of erythropoietin in the human body is a special type of fibroblast cell neighbouring blood capillaries throughout the kidney’s cortex. It might seem odd that the body uses the kidneys as one of its oxygen sensing organs, but the cortex of the kidney is an ideal place to measure the blood’s ability to deliver oxygen, because it’s one of the few tissues in the body where blood flow to capillaries is kept almost constant.
Calcitriol (1,25 dihydroxyvitamin D)
You’ve probably heard of vitamin D, the “sunshine vitamin”: Ultraviolet light is needed in the chemical reactions that convert sterols – usually cholesterol – into vitamin D. In humans, this happens in the skin, although people living indoors, or far from the equator, or in cloudy climates have to get their vitamin D from their diet.
The role of vitamin D in the body is to serve as the raw material to make the hormone calcitriol (1,25 dihydroxyvitamin D). The main function of calcitriol is to tell the intestine to increase the absorption of calcium and phosphate from food. When blood calcitriol levels are low, the gut absorbs less than 1/6 of the calcium in the food; When blood calcitriol levels are high, the gut absorbs most of the calcium in food.
In calcitriol production, the first hydroxylation step happens in the liver. The second hydroxylation step, converting the precursor (or prohormone) to its active form, calcitriol, happens in the proximal tubule cells of the kidney. Importantly, the second hydroxylation step is much slower than the first, so the rate-limiting step for calcitriol production happens in the proximal tubule cells of the kidney. This allows the kidney to control calcitriol levels in the blood.
The proximal tubule cells vary the second hydroxylation step in response to three signals:
- calcitriol production increases in response to parathyroid hormone,
- calcitriol production decreases in response to low blood calcium levels, and
- calcitriol production decreases in response to low blood phosphate levels.
Thus, the kidney integrates signals and communicates with the gut to keep calcium and phosphate levels in the blood constant.

Renin
In addition, the kidney secretes the hormone renin. Renin is an unusual kind of hormone, because its signalling mechanism is unique: Renin is an enzyme, specifically a protease. This enzyme hydrolyzes the circulating precursor angiotensinogen into angiotensin I, which is further converted in the lungs to the vasoactive peptide angiotensin II. You can see why renin is also called angiotensinogenase.
The pericyte cells of the juxtaglomerular apparatus are the site of renin production and release. The kidney responds with secretion of renin when the juxtaglomerular apparatus detects:
- a decrease in arterial blood pressure,
- a decrease in extracellular fluid sodium, or
- a stimulus by the sympathetic division of the autonomic nervous system that controls blood pressure through the β1 adrenergic receptors.
The angiotensin produced by renin’s proteolytic activity has several downstream effects:
- increases Na+ reuptake in the kidney’s proximal tubules,
- constricts most arteries and veins, increasing the blood pressure,
- increases the glomerular filtration rate by constricting the efferent arteriole from the glomerulus more than the afferent arteriole to the glomerulus,
- stimulates the posterior pituitary to release antidiuretc hormone (vasopressin), which causes collecting duct cells to become permeable to water, allowing the renal medulla to retreive water from the urine,
- stimulates the adrenal cortex to release aldosterone, stimulating the kidney to retain sodium while excreting potassium, and
- acts on the brain to stimulate thirst for water and appetite for salt.
Notice that many of these effects allow the kidney to control the volume of the extracellular fluid, including blood plasma, lymph, and interstitial fluid. This is largely accomplished by by retaining sodium in the extracellular fluid, thereby increasing osmotic pressure.

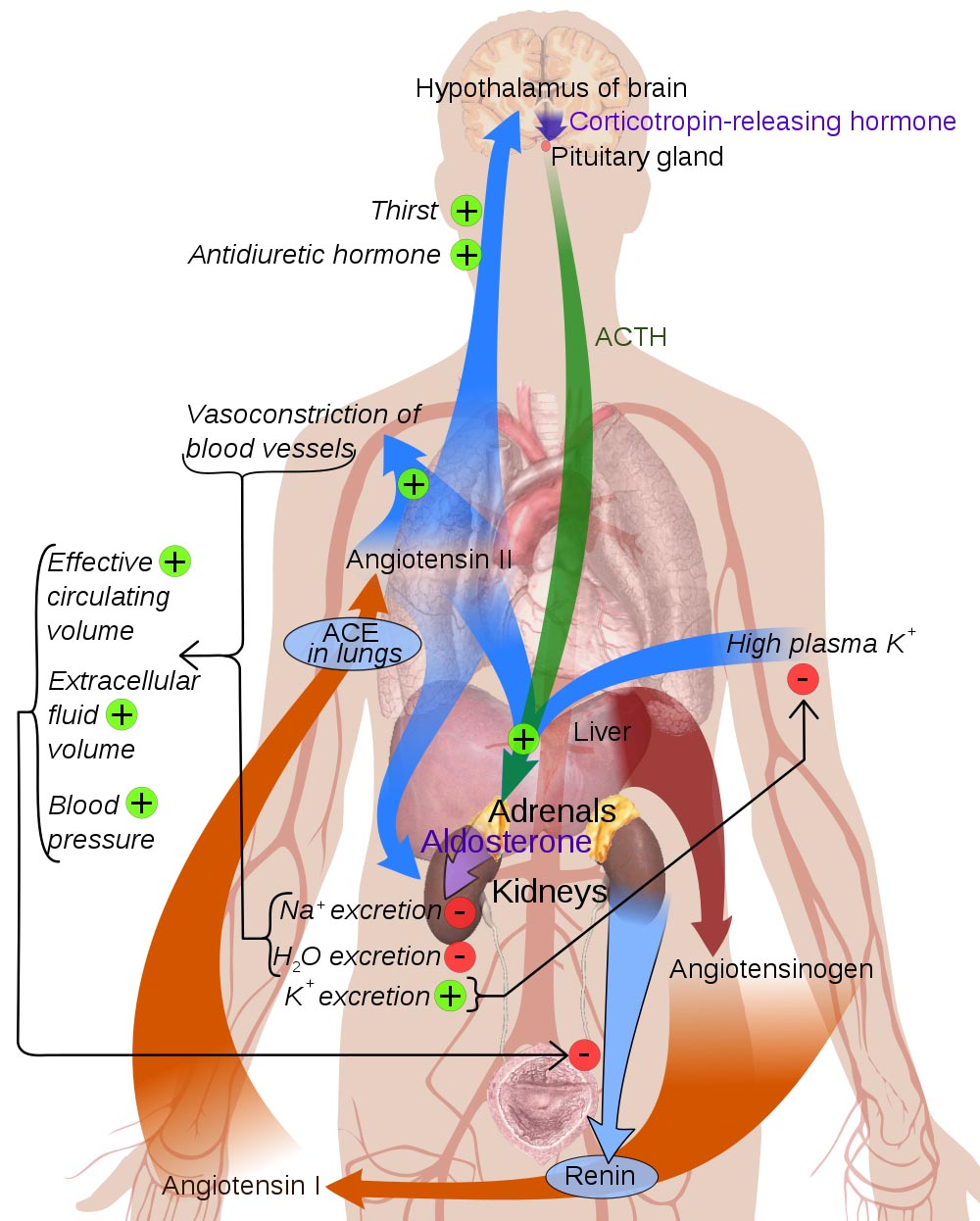