Digging In
3 Soil Organic Matter
Sylvie Quideau; Myrna Simpson; and Adam Gillespie
LEARNING OUTCOMES
On completion of this chapter, students will be able to:
- Define soil organic matter and list its main components
- Evaluate the importance of organic matter on soil physical, chemical and biological properties and processes
- Explain the sources and turnover processes of soil organic matter
- Discuss the spatial distribution and land management of soil organic matter
- Critically evaluate how soil organic matter is measured and quantified
- Recognize the role of soil organic matter in global issues
INTRODUCTION
Soil organic matter has been increasingly in the news lately, in part because the vast majority of terrestrial carbon is contained in soils. As such, soils play an important role in the global carbon cycle. In addition to serving as a key carbon store, soil organic matter is one of the central attributes of soils. Organic matter influences virtually all of soil properties and overall health, including its physical structure, nutrient status, and biodiversity. Soil organic matter is composed of plant and animal residues at various stages of decomposition, microbial biomass, and products of microbial synthesis. Organic matter accumulation in soils reflects the balance between plant litter inputs and losses through microbial respiration, erosion, and leaching. Carbon stocks may vary widely among soils and across the landscape as a function of parent geological material, climate, vegetation, and topography.
WHAT IS SOIL ORGANIC MATTER?
Soil organic matter is comprised of both living and non-living components. The living component includes soil macro- and micro-fauna, and soil microbial communities, which may be active or dormant. The non-living portion of soil organic matter is derived from dead plant and faunal inputs into the soil (Figure 3.1). These inputs undergo various biogeochemical processes and are either lost, preserved or transformed in soil. The non-living component of soil organic matter is currently hypothesized to be a complex mixture of different biologically-derived molecules that are at various stages of oxidation and preservation (Schmidt et al., 2011). The chemical structure of soil organic matter has been under debate for many decades and it was previously believed that the non-living component of soil organic matter was made of recognizable plant- or animal-derived biomolecules and humic substances, large by-products that resulted from both biological and chemical decay of organic inputs to soil (Schmidt et al., 2011; Lehmann and Kleber, 2015; Can You Dig It Box 1). A more current paradigm recognizes the key contribution of microbial communities in the formation of soil organic matter (Simpson et al., 2007). As organic inputs are decomposed and processed by microorganisms, the majority of the carbon is respired as CO2, but some is incorporated into microbial biomass, can interact with clay minerals or is partially degraded and remains in the soil. Consequently, soil organic matter consists of a complex mixture of plant, animal or microbial-derived residues at various stages of decomposition, and of new molecules synthesized by microbes. Soil organic matter can also interact with soil minerals to form organic matter – mineral complexes that contribute to soil aggregation and may increase the stability of organic matter in soil by hindering microbes from physically accessing organic matter substrates that are protected through these organic matter – mineral interactions (Oades, 1988; Baldock and Skjemstad, 2000).
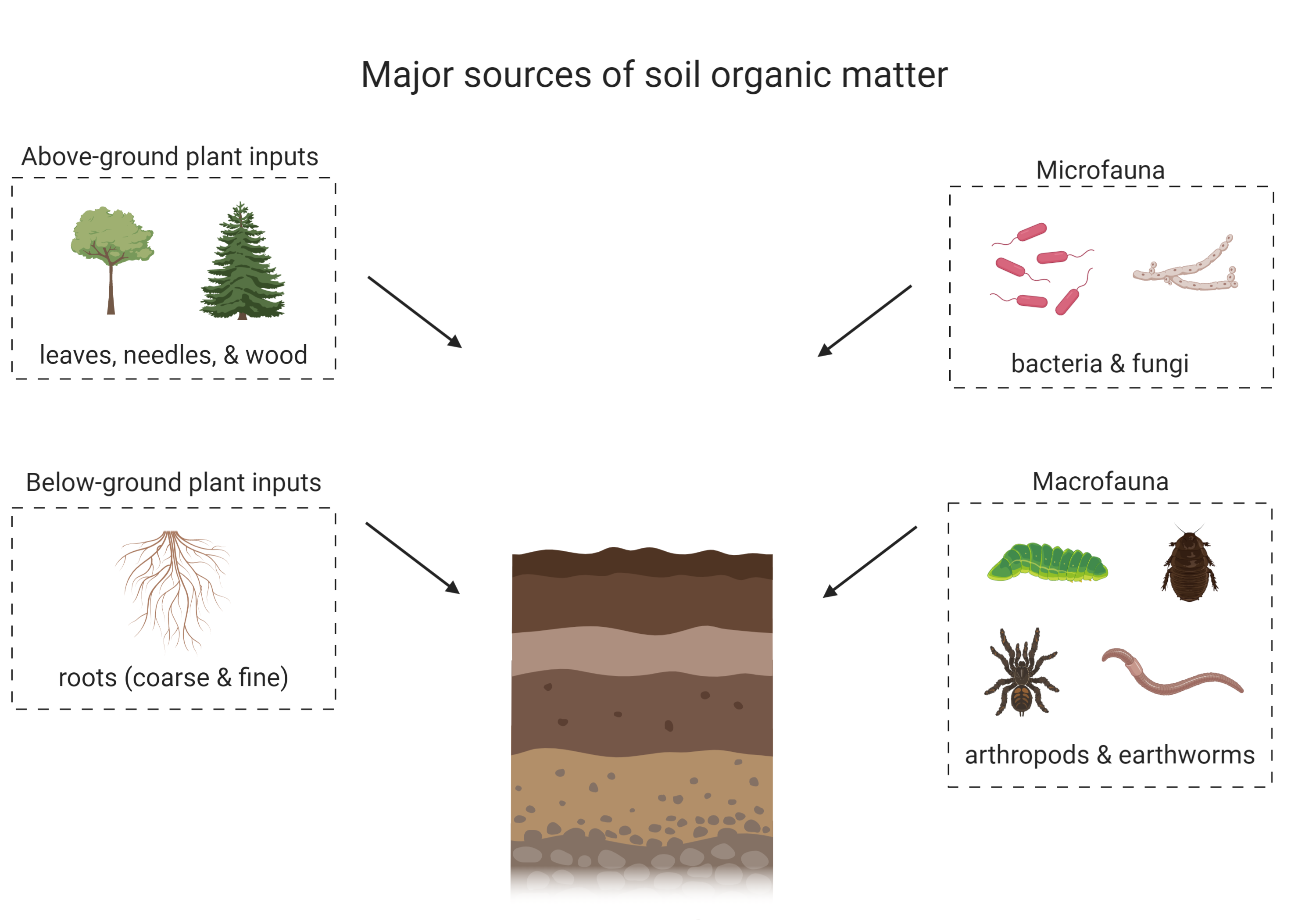
Organic matter plays several key roles in soil properties and soil processes. The presence of organic matter improves soil stability by promoting aggregation which reduces the potential for soil erosion. Soil organic matter also improves water retention in soil and has a high cation exchange capacity (from 100 to 500 cmol kg-1) which contributes to the total cation exchange capacity of the soil, depending on the amount of soil organic matter present. This improves the soil’s ability to retain important cations (Ca2+, Mg2+, K+, and Na+), that can enhance the buffering capacity of the soil. Soil organic matter is also the most biogeochemically active and dynamic portion of the soil and it is important for regulating many processes related to the global cycling of elements such as carbon. Soil organic matter acts as a storehouse and a slow release fertilizer for many plant nutrients, including nitrogen, phosphorus and sulfur. Finally, organic matter supports a large and varied faunal and microbial community.
WHY IS SOIL ORGANIC MATTER IMPORTANT?
Soil organic matter is intimately linked to numerous key physical, chemical, and biological soil properties. It is so important for soil function that it is almost impossible to find a soil property that is not influenced by soil organic matter in some way. Throughout this textbook, every chapter refers to how soil organic matter is influential on the overall expression of soil physical, chemical and biological properties. Table 3.1 shows many of the important components that soil provides to crop production, and how soil organic matter influences these components. In many of these instances, we can see that the properties of soil organic matter allow it to mitigate plant growth limiting conditions and accentuate important plant growth-promoting process in soils.
Soil Water: Soil organic matter has the ability to buffer water supply in soil. High organic matter increases water infiltration during intense rain events, limiting ponding, soil saturation and anoxic conditions which limit plant growth. Soil organic matter also has the ability to retain water in times of drought and water deficit. Together, these two phenomena act to stabilize the hydrodynamics of plant water supply. Sandy soils benefit hydrologically from increased organic matter because they are intrinsically droughty as a result of large inter-particle pore spaces that do not hold water tightly.
Soil Structure and Compaction: Soil aggregation is a strong indicator of healthy soil structure because it reduces stress on root growth and allows for better water infiltration. Indeed, granular structure tends to be encouraged in soils with higher organic matter, whereas soils exhibiting management-induced blocky structure also tend to have shown soil organic matter depletion. Compaction, which is always a risk in heavily managed soils, can be mitigated in soils with higher organic matter levels, particularly in the particulate, or ‘light’ organic matter fraction (see Soil Organic Matter Composition section). Finally, clay soils can be more easily managed when organic matter is high because it reduces the inter-particle cohesion and plasticity.
Soil nutrient status: Organic matter contains a wide range of functional groups that can increase cation exchange capacity. Indeed, soil organic matter may contain between 50 to 90% of the cation-adsorbing ability of a soil. Also macronutrients of nitrogen, phosphorus and sulphur have very important storage pools in soil organic matter, becoming available to plants through microbial mineralization.
Soil cation exchange capacity: Soil organic matter contributes to the total cation exchange capacity of soil. Soil organic matter cation exchange capacity ranges from 100 to 500 cmol kg-1 and is considerably higher than most minerals found in soil. Soil organic matter has a pH-dependent cation exchange capacity due to the dissociation of its functional groups with increasing pH of the soil solution. Cation exchange capacity is important for the ability of a soil to retain important nutrients, which include the major cations Ca2+, Mg2+, K+, and Na+ that also contribute to the buffering capacity of the soil.
Soil biology and energy storage: Chemical bonds in soil organic matter contain energy, and this energy is consumed by microbes for growth and metabolism. It is this stored energy that drives microbial nutrient cycling and all the other plant-beneficial associations that soil biology maintains with plants.
Table 3.1. Contributions of soil organic matter to fundamental soil properties
Function component | Function characteristics/processes | Effect of Soil Organic Matter |
---|---|---|
Soil Structure | Aggregate stability | Promotes formation of stable soil aggregates |
Compaction | Particulate organic matter helps resist compaction | |
Erosion | Aggregate stability helps reduce erosion | |
Crusting | Helps reduce preconditions to crust formation (i.e., erosion and aggregate destruction) | |
Nutrient cycling | Accept, hold, and release cations | High cation exchange capacity of soil organic matter improves the ability to retain base cations |
Oxy-anion supply (N, P, S) | Organic N, P and S pools are source of plant available macronutrients via microbial mineralization | |
Regulate water | Infiltration and retention | Encourages stable and divers pore structures to receive, store, and release moisture for plant use |
Water supply | Adequate water retention to buffer and reduce effects of drought | |
Buffers extreme rainfall events | Encourages infiltration to reduce erosion and pooling in extreme rain events | |
Microbial function | Microbial diversity | Wide range of substrates supports many metabolic pathways, encouraging microbial diversity |
Store and release (recycle) energy | Primary repository of chemical energy used in microbial respiration, drives all major nutrient cycles | |
Plant growth promotion | Supports higher levels of microbes which may benefit plant growth through disease suppression. | |
Medium of plant growth | Seed germination and root growth | Improves seed imbibement and access to nutrients, reduces physical force on growing roots |
Buffer acidity, sodicity | High CEC of soil can buffer and reduce adverse chemistry | |
Waste management | Sequester biotoxic elements | High CEC can reduce metal mobility |
Degrade organic substances | Supports diverse microbial population that can degrade xenobiotics |
WHERE DOES SOIL ORGANIC MATTER COME FROM AND WHY DOES IT PERSIST?
Carbon Inputs to Soils
Soil organic matter can have a diverse range of inputs, which are preserved or transformed through various biological and chemical reactions to form soil organic matter (Schmidt et al., 2011; Kögel-Knabner and Amelung, 2013). Some inputs can also be stabilized in the soil (in the form of soil organic matter or through interactions with clay minerals; Oades, 1988; Kleber et al., 2007) or destabilized and leave the soil through erosion or export with water (Bailey et al. 2019). Soil inputs vary with ecosystem properties and land management practices but predominantly include necromass from soil animals (macro- to micro-scale) and above- and below-ground plant litter (Schmidt et al., 2011; see Figure 3.1). Some soils may also receive inputs such as pesticides, herbicides, fertilizers, and biochar/charcoal from processes related to land management and other anthropogenic activities. Plant inputs are believed to be a major contributor to soil organic matter (Kögel-Knabner, 2002), but some studies suggest that microbial sources are underestimated (Simpson et al., 2007). The nature and quantity of soil organic matter inputs depends on the soil environment as well as ecosystem management. Another consideration is that soil organic matter composition is closely related to soil development (see Chapter 2 for more information) as younger soils may not have as much soil organic matter as older soils which have developed a thick O or A horizon over time, depending on the soil and environmental properties. For example, Chernozemic Ah horizons are more enriched in soil organic matter, especially Black Chernozems which are found in cooler climates. These ecosystem conditions encourage soil organic matter accumulation. In contrast, Ap horizons contain less soil carbon than their native counterpart (Ah) because of agricultural land use. As well, organic matter accumulates in peatlands due to high water saturation and anaerobic conditions that limit microbial activity.
The key factors that control soil formation (abbreviated as clorpt) are: climate, organisms (biota), relief (topography), parent material, and time (Jenny, 1941). These also play a role in soil organic matter formation and biogeochemistry. The composition (quality) and amount (quantity) of inputs will vary with different ecosystems and this is controlled by soil forming factors such as climate. Climate also determines the types of biota and also, their activity. Topography can determine the availability of oxygen which is required for the aerobic degradation of inputs as well as stored soil organic matter. Topography can also mediate soil water content, which is required for plant growth. Moisture content may also limit microbial decay of plant inputs and enhance organic matter accumulation, such as in wetlands. The parent geologic material and formation of secondary minerals is also important for soil organic matter because clay minerals can bind soil organic matter which increases soil organic matter stabilization over time.
Climatic conditions such as mean annual temperature and mean annual precipitation are important for determining soil inputs and soil organic matter processes over both short and long-time scales. For instance, temperature and moisture will control the types of plants that inhabit an ecosystem and cooler climates favour coniferous-dominated versus deciduous-dominated trees in forests. The types and quantity of shrubs and grasses that are present are also determined by climate and other soil properties. Figure 3.2 shows the ranges of mean annual temperature and precipitation for different biomes. These parameters also contribute to the quantity and quality of inputs to soil and the extent to which they are transformed as warmer climates favour soil organic matter degradation (through microbial respiration) but also encourage plant growth. Cooler climates may limit plant growth but can also restrict microbial degradation and bioturbation by macrofauna.

As described previously (see Figure 3.1), there are varying types of inputs that enter soil and some are preserved via sorptive interactions with clay minerals and some are degraded by native soil biota. The chemistry of soil organic matter inputs has been studied for several decades to help understand soil organic matter formation processes as well as predict soil organic matter stability. The major types of inputs and their sources are summarized in Table 3.2.
Table 3.2. Major soil inputs and their properties that contribute to soil organic matter formation and persistence (Kögel-Knabner 2002; Schmidt et al. 2011; Kögel-Knabner and Amelung 2014)
Input | Source(s) | Chemistry | Stability |
---|---|---|---|
Lipidsa | Plants and microbes | Small molecules | Varies (low-high) with carbon chain length and functional group |
Proteins/peptidesb | Plants and microbes | Macromolecules | Medium |
Cellulose (hemicellulose) | Plants and microbes | Macromolecule | Low |
Lignin | Plants | Macromolecule | Medium-high |
Chitin | Fungi & Arthropods | Macromolecule | Medium-high |
Cutin | Plants (waxy coating on leaves) | Macromolecule | High |
Suberin | Plant roots | Macromolecule | Low-High |
Char/biochar | Pyrolyzed plant material | Macromolecule | High |
aLipids are a wide range of compounds that have ranges of carbon lengths as well as functionalities. Some compounds in this group have alcohol or acid functionalities. | |||
bThis encompasses a large group of molecules that have varying chemical properties and stability |
Degradation of Carbon Inputs
Soil organic matter inputs (Table 3.2) have varying chemistry, which can contribute to their susceptibility for microbial degradation after being introduced into the soil environment. Some inputs are degraded more rapidly than others. Many of the inputs are large molecules (macromolecules) that decompose in stages. For example, cellulose is a complex carbohydrate but once its macromolecular structure is broken down, it produces simple sugars that can be readily degraded. Lignin, which is another macromolecule composed of a network of aromatic (phenolic) components, is more difficult to break down and is primarily degraded by fungi. Therefore, decomposition of plant inputs is a function of the chemistry of the inputs, the availability of microbes and enzymes, as well as nutrient content, and other environmental factors (such as oxygen and water).
Under aerobic conditions, organic matter inputs are degraded via respiration (Equation 1.3). Respiration, also referred to as mineralization, is an oxidation reaction where oxygen acts as the primary electron acceptor (Bohn et al. 1985). The net products of this reaction (Equation 1.3) are carbon dioxide and water. As shown, a basic form of organic matter inputs (CH2O) yields 4 electrons (Equation 1.1), which then require the equivalent amount of oxygen (Equation 1.2) to balance the net reaction (Equation 1.3):
CH2O + H2O → CO2 + 4e– + 4H+ (1.1)
O2 + 4e– + 4H+ → 2H2O (1.2)
CH2O + O2 → CO2 + H2O (1.3)
More complex forms of organic matter require more chemical energy for degradation (Bohn et al. 1985). For example, if empirical formulae are considered for both lignin (C2.9H2.9O) and cellulose (C1.2H2O), it is evident that the degradation of plant material (estimated as two-thirds cellulose and one-third lignin for the purposes of this example; Equation 2.1) will require less chemical energy and less oxygen (which acts as a terminal electron acceptor) than soil organic matter (empirically represented as C2.2H2.2O; Equation 2.2). This requirement is one reason why more complex forms of organic matter are stabilized over other forms and persist longer in soil (Table 3.1).
Plant matter decomposition: C1.7H2.2O → 1.7C4+ + H2O + 7e– + 0.2H+ (2.1)
Soil organic matter: C2.2H2.2O → 2.2C4+ + H2O + 9e– + 0.2H+ (2.2)
Inputs from plants and microbes are respired to CO2 based on oxidation as shown in Equation 1.3. But the rate at which respiration occurs is dependent on ecosystem properties, microbial biomass and diversity, and nutrient availability. Figure 3.3 shows the fate of fresh plant material in the soil carbon cycle. The addition of fresh plant residues often fuels microbial activity (referred to as soil priming; Kuzyakov et al. 2000), which can then result in continued degradation of stored soil carbon based on energetics described in Equations 2.1 and 2.2. This may then in turn destabilize stored soil carbon and reduce soil carbon stocks. In some cases, fresh plant material decomposition may also increase soil carbon stored (recall, soil carbon includes both the living and non-living components). Therefore, how fresh plant additions alter soil carbon can vary but this is dependent on the quality of the inputs, presence of microbial degraders and enzymes, oxygen and water content, and time. In the example highlighted in Figure 3.3, fresh plant carbon is partly respired to CO2 and also stored in the form of soil carbon. The total amount of carbon doesn’t change (carbon balance exists) but the form of the different carbon pools (atmospheric CO2, soil organic matter, soil microbes, etc.) varies with time, space and ecosystem properties. For example, this process may occur more quickly in aerobic soils in warmer regions of the world. In other ecosystems, the time required for plant carbon to be converted into other carbon forms can be much longer (several decades at least). Under anaerobic conditions, the process of respiration (conversion of plant carbon to CO2) is much slower due to the limited availability of O2 (see Equation 2.1). Consequently, the time scale for plant carbon conversion into other forms varies with climate and other ecosystem properties, as well as the quality of plant carbon.

Can You Dig It!
Humic substances

It was historically believed that the long-term stability of soil organic matter was due to the conversion of plant and animal inputs into humic substances through different biological and chemical reactions. The traditional fractionation method used to isolate different components of soil organic matter (Schmidt et al. 2011; Lehmann and Kleber 2015) uses a series of strong bases and strong acids to separate out soil organic matter based on its solubility (see side Panel A).
Part of the soil organic matter was not extractable in either strong base or strong acid. The resulting fractions were then characterized to learn more about soil organic matter composition (see side Panel B). These humic substance fractions (fulvic acid, humic acid and humin) were historically analysed using elemental analysis and other low-resolution chemical analysis methods. This resulted in soil organic matter scientists interpreting that these fractions were of high molecular weight (large macromolecules). It was then believed that these large macromolecules were stable in soil in the long-term and the main process by which soil inputs are stabilized in soil environments.
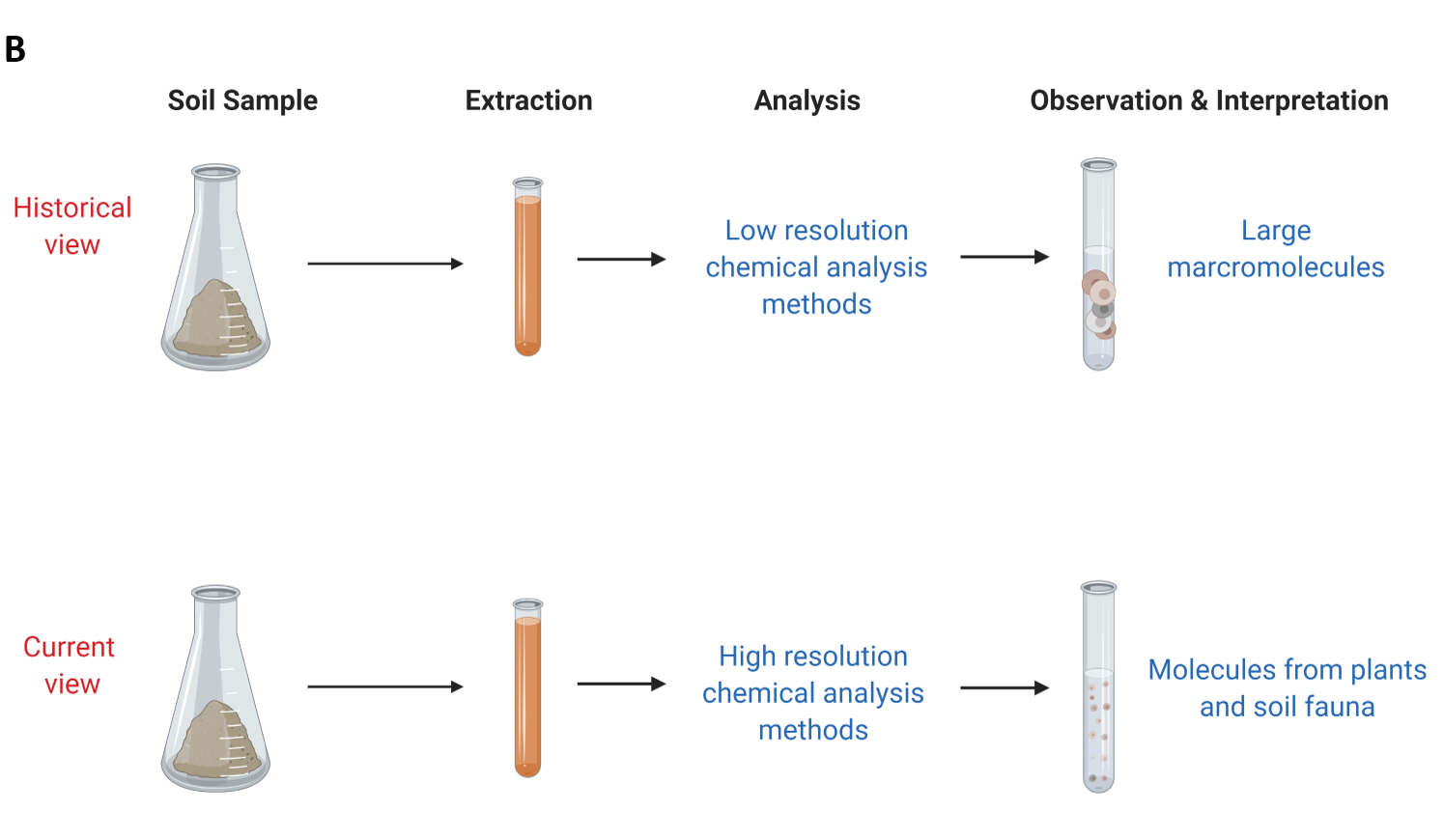
Starting in the late 1990s, the advancement of chemical analysis methods allowed scientists to obtain high resolution data about the chemistry of soil organic matter (Kelleher and Simpson 2006; Schmidt et al. 2011). These methods showed that soil organic matter was actually made of recognizable biomolecules from plants and animals at varying stages of degradation (see Panel B). These methods also showed that these biomolecules can associate with each other and behave like larger molecules (consistent with the historical view). Scientists are further exploring the current view and how this new information can be used to predict and explain the stabilization of soil organic matter in different environments and with climate change.
Persistence of Soil Organic Matter
Many inputs enter soil and are transformed into soil organic matter or mineralized to CO2. But some soil organic matter can reside in soil for decades to hundreds of years. Part of this long-term persistence is due to the strong interaction between soil organic matter and clay minerals found in soil (Oades 1988; Schmidt et al. 2011; Simpson and Simpson 2012; see Figure 3.4). Clay minerals can stabilize soil organic matter through the formation of organic matter-mineral complexes in soil (Figure 3.4b). Both amorphous and crystalline clay minerals can bind soil organic matter but to varying extents and via different chemical interactions such as covalent, ionic or weak van der Waals interactions (Feng et al. 2005; Kleber et al. 2007). Although the manner in which soil organic matter interacts with clay minerals differs in each soil environment, it is clear that the binding of organic matter to clay minerals increases its persistence (Baldock and Skjemstad 2000). Once bound to clay minerals, soil organic matter is less available for biological degradation because microbes cannot gain easy access to organic matter sequestered on clay surfaces. As such, soil organic matter bound to clay minerals is more persistent in the long-term as it is not as readily used as a microbial substrate. Other mineral surfaces that facilitate organic matter persistence are iron and aluminum oxides in acidic soils. In neutral and alkaline soils, the presence of polyvalent cations such as calcium and magnesium stabilizes organic matter by forming bridges between negatively charged organic molecules and clay surfaces. The type of clay minerals is also important in controlling how much carbon can be ultimately stored in a soil. Clay minerals with higher surface areas (2:1 clays) have a higher ability to adsorb carbon than 1:1 clays. In Canada where soils are still relatively young, clay mineralogy mostly reflects that of the geological parent material.

In addition to the interaction of organic matter with mineral surfaces, physical protection inside aggregates (Figure 3.4a) is considered to be an important mechanism for organic matter persistence (Six et al. 2002). In that case, organic matter mostly persists in soils because it is spatially separated from the decomposing microbial communities. Furthermore, the lower availability of oxygen within aggregates may become a limiting factor to microbial activity. In boreal ecosystems, which cover the majority of Canada’s land area, fire is a recurrent disturbance that produces pyrogenic carbon, often referred to as black carbon or char. Some pyrogenic carbon, containing highly condensed aromatic structures, may persist in soils and constitute a long-term carbon sink (Ohlson et al. 2009). However, the persistence of pyrogenic carbon in soils greatly varies depending on its formation conditions (Soucemarianadin et al. 2015)
Traditional views highlighted the importance of organic matter chemical structure in explaining its persistence in soils. A newer paradigm questions the validity of this assumption and suggests instead that molecular structure alone does not explain organic matter accumulation and stability in soils. In addition, microbial metabolism, and more specifically carbon utilization efficiency plays a role in determining organic matter persistence (Cotrufo et al. 2013). Under this new model (Figure 3.4c), it is proposed that more labile molecules, because they are preferentially taken up by microbes hence retained with the soil, constitute the main portion of stable soil organic matter.
HOW CAN WE MANAGE SOIL ORGANIC MATTER?
Environmental Factors
Soil carbon storage results from the balance between carbon inputs and outputs. Carbon sequestration occurs when aboveground and belowground (i.e., leaf and root-derived) inputs exceed outputs, which are usually dominated by CO2 fluxes from the soil surface, but may also include in some instances important contributions from methane (CH4) and leaching of dissolved organic carbon (DOC). Plant residues are the major substrate for soil organic matter formation. When microbial communities decompose plant litter, the majority of carbon contained in these residues is eventually evolved as CO2. However, a small portion remains in the soil as soil organic matter, sometimes for centuries or even millennia. Figure 3.5 shows the distribution of soil carbon (related to soil organic matter) of the soils of Canada. The intricate balance of these inputs and outputs, as well as organic matter stabilization to clay minerals, is what determines soil organic matter persistence.

The rate of carbon accumulation varies greatly among soils, which reflects the influence of environmental factors; i.e., soil forming factors (climate, organisms, parent geological material, relief and time) on pedogenic processes. Climate directly affects soil carbon stocks by controlling the balance between litter inputs and decomposition outputs. Increasing precipitation increases plant growth and litter production, and decreasing temperature decreases decomposition losses. At the global scale, carbon accumulation in soils is more influenced by temperature than precipitation and tends to increase in high-latitude soils where low temperatures limit decomposition. The largest stocks of carbon are found in the northern permafrost region, where both Organic soils and Cryosols are present. In arid environments, water availability may limit plant growth and plant residue inputs to soils, and consequently lead to lower soil carbon stocks.
Across Canada, soil carbon stocks also vary as a function of precipitation, where high local precipitation along both the Atlantic and Pacific coasts allows for the establishment of dense forest vegetation and consequently, large surficial litter inputs. In addition, placement of the vegetation inputs directly affects the distribution of carbon within the soil profile as well as total soil carbon stocks. In grassland soils where belowground (root) inputs can be plentiful, carbon stocks can be greater and not decrease as sharply with depth as they do under forest vegetation. Lastly, while vegetation may be the main factor controlling surface organic carbon, the soil parent geological may also be a key factor for carbon sequestration in the mineral soil profile. Soil carbon and clay content are positively correlated, both because of the formation of organic matter-mineral complexes resistant to decomposition losses, and because of the indirect effects of clay that promotes greater fertility and plant growth, hence increases carbon returns to the soil.
At the landscape level, organic carbon accumulation is favoured in lower slope positions where soil conditions are wetter and tend to inhibit decomposition losses. Similarly, in the northern hemisphere, soil carbon stocks tend to be greater on north-facing slopes compared to south-facing slopes, where lower temperature and lower evaporation increase soil moisture and decrease decomposition.
Average carbon stocks in the different mineral soil orders found in Canada vary from around 11-12 g m-2 for Regosols, Brunisols, Luvisols, Chernozems and Solonetzs to 16-20 g m-2 for Podzols and Gleysols. In forest soils, carbon stocks increase from well-drained Luvisolic and Brunisolic soils to poorly drained Gleysolic and Organic soils (Figure 3.6). Carbon stocks in Organic soils may be an order of magnitude higher than in other soil types and average 134 g m-2. Within mineral soil orders, Cryosols contain the highest stocks averaged at 41 g m-2. Cryosols also occupy the largest area in Canada, as they constitute more than one third of Canadian soils. Cryosols and Organic soils contain roughly the same amount of carbon, which is by far the largest amount compared to other soil orders. Taken together, these two soil orders hold about 80% of the total carbon stocks contained in Canadian soils. Podzols and Brunisols contain 5-10%, and the remaining orders < 5%.
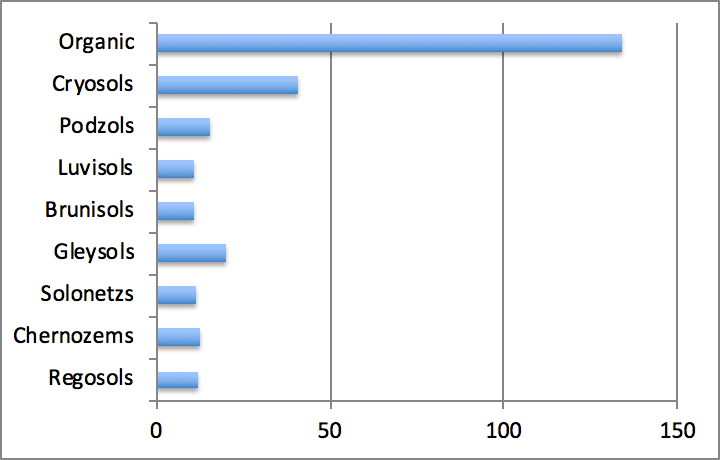
Pedogenic processes (i.e., the processes of soil formation) can directly affect carbon stocks and carbon stability in soils. In turn, soil carbon content and soil classification are directly related for many soil types. The most obvious example may be the case of Organic soils, where organic matter accumulation is at the core of their formation and classification. Paludization, the accumulation of thick organic layers on the mineral soil surface, creates the fibric (Of), mesic (Om), and humic (Oh) horizons representative of Organic soils. In upland forests, littering, the thinner, surficial accumulation of fresh plant residues, is responsible for forest floor accumulation, which can be separated into three distinct horizons (LFH) based on the degree of decomposition (see Chapter 8 for further details).
Humification, the formation of humic substances from plant residues, and melanization, the darkening of a soil color due to carbon addition, are both responsible for the accumulation of carbon within the top mineral soil layers, in particular in Chernozemic horizons. The formation of these horizons is favoured by the extensive network of grass roots that contributes large exudate and residue inputs. Bioturbation by earthworms and stabilization of organic matter by calcium are two common processes contributing to carbon accumulation in Canadian Chernozems. The extent of darkening is used to differentiate between great groups of Chernozems and Solonetzs, with in increasing amount of carbon: Brown, Dark Drown and Black great groups. The absence or presence of a carbon-rich Ah horizon is also used to differentiate great groups of Brunisols, Luvisols, Gleysols, and Regosols; i.e., Melanic and Sombric Brunisols, Gray Brown Luvisols, Humic Gleysols and Humic Regosols. Lastly, carbon content is used to separate subgroups of several additional mineral soil orders. For instance as opposed to the processes just mentioned, podzolization results in carbon accumulation at depth. Mostly, during podzolization, carbon is added from soluble organics leached from the soil surface. The other carbon input at depth would result from root exudates or root turnover. Podzolic great groups are partially differentiated based on their carbon content, with the Ferro-Humic Podzols containing more carbon in their subsoils than the Humo-Ferric Podzols.
Lastly, processes that are not directly linked to humification may nonetheless increase soil carbon. Gleization, which results in anaerobic conditions, may lead to the accumulation of carbon in Gleysols. Extreme cold, which also inhibits decomposition, can result in thick surficial carbon accumulation characteristic of Organic Cryosols, and, in the case, of Turbic Cryosols, in carbon accumulation in the mineral horizons, which can also be found at depth due to cryoturbation, the process of soil mixing due to freezing and thawing.
Land Management
In Canada, conversion of native land to arable agriculture resulted in a loss of about 25% of the carbon present in the soil surface layers (0-30 cm) prior to the onset of cultivation. In other words, carbon lost over the 19th century from Canadian cultivated grassland soils would have resulted in an emission of about 1 Pg (Janzen et al. 2018).
Carbon losses may be attributed to removal of crop residues, as well as to increased erosion and decomposition. In some cases, fire was used to clear land, which resulted in increased losses. Increased decomposition, rather than erosion is typically he main culprit following cultivation, and results from the physical disruption of soil aggregates by tillage, which exposes previously inaccessible organic matter to the degradative actions of soil microorganisms. The majority of carbon lost upon cultivation consists of the light fraction, which is composed of still “young”, only partially decomposed plant residues. Most of the loss occurs rapidly, i.e., during the first twenty years of soil conversion to arable agriculture.
Because of past losses of carbon from agricultural soils, these have significant capacity to store more carbon. Several management practices can promote soil carbon gains. Specifically, some can decrease soil carbon losses including: reduction in tillage intensity and reduction in summer fallow. Some measures contribute by increasing carbon inputs to soils, either by adding carbon from other sources (e.g. manure, biochar), or by promoting greater plant inputs to soils (e.g., by growing cover crops, improving residue retention, increasing use of perennials, and optimizing fertilization). Chemical fertilization (i.e., addition of inorganic fertilizers), by promoting plant growth, typically results in carbon accumulation in soils. The annual carbon storage rate for soils receiving inorganic fertilizers has been estimated at 23 ± 13 g C m-2 yr-1 (VandenBygaart et al., 2004). Application of organic amendments such as animal manure or biosolids (human waste) also leads to an increase in soil carbon, especially when applied regularly over many years or even decades. However, this may also increase the generation of greenhouse gases, in particular nitrous oxide. An upper limit in soil carbon sequestration may be reached, which corresponds to the fact that the soil matrix may become saturated with carbon as it has reached its full storage capacity. Fine-textured soils have a higher ability to store carbon than coarser-textured soils.
Conservation tillage, including reduced and no tillage, can promote soil carbon accumulation, although recent studies have shown that the increase is likely confined to the upper layer of soil (0-10 cm), and that tillage has no effect on carbon stocks integrated over the full 0-130 cm depth interval (Mary et al., 2020).
Soil organic matter can also be impacted by other anthropogenic activities. It is well documented that conversion of native lands for use in farming can lower soil organic matter content, especially with tillage. The incorporation of crop residues, as well as conservation tillage practices, is being used to preserve soil organic matter in agricultural lands. Crop residues are applied to the soil surface after harvest and are reincorporated into the soil organic matter pool. The application of charred residues (biochar or through slash and burn agriculture) improves both the organic matter content and cation exchange capacity of the soil and alters the soil pH, all of which benefits both plants and soil microbes. Char can be long-lived in soil, and though the benefits of its application vary with time as the composition of the char can change, the addition of char does contribute to the overall soil organic matter continuum found in managed soils.
While deforestation typically results in a loss of soil carbon, afforestation may, at least in part, restore the original soil carbon levels. Reforestation in the boreal zone results in smaller rates of soil carbon increase compared to other climates, likely because tree growth rates are slower there (Laganière et al., 2010). Previous land use and the type of disturbance is also an important factor controlling the rate of soil carbon increases following afforestation. Afforestation of agricultural lands yields to greater increases in soil carbon stocks compared to pastures or grasslands (Mayer et al., 2020). Afforestation of soils disturbed by industrial activities, such as surface mining, can also lead to a significant increase in soil carbon levels (Prescott et al., 2019). If suited to the site conditions, deciduous trees help to increase soil carbon stocks faster than conifers do; in the long run, deciduous trees also contribute to higher carbon stocks in the mineral soils compared to the forest floors.
Table 3.3. Effects of land use changes on soil organic carbon stocks. Compiled from VandenBygaart et al. (2004); Horwath and Kuzyakov (2018); and Janzen et al. (2018)
Management practice | Location | Carbon storage rate | Δ soil carbon |
---|---|---|---|
(g C m-2 yr-1) | (%) | ||
Grassland conversion to agriculture | Eastern Canada | -30 to -36 | |
Grassland conversion to agriculture | Western Canada | -11 to -59 | |
Forest conversion to agriculture | Eastern Canada | -4 to –49 | |
Forest conversion to agriculture | Western Canada | -11 to –36 | |
Overall conversion to agriculture | Canada | -24 ± 6 | |
Organic amendments | World | + 40 to 62 | |
Chemical fertilizers | Canada | + 23 ± 13 | |
Chemical fertilizers | World | + 16 to 32 | |
Chemical fertilizers + organic amendments | World | + 33 to 52 | |
Conventional to no tillage | Canada | + 5 to 16 | |
Cover crops | World | + 15 to 23 | |
Afforestation of agricultural soils | World | + 9 to 51 |
HOW DO WE MEASURE SOIL ORGANIC MATTER?
There are two major categories of soil organic matter analysis: total elemental analysis; and chemical speciation. Both groups of analyses are important for answering a range of environmental and agronomic questions. Importantly, soil organic matter is related to soil organic carbon but these are not the same measurement. This is an important distinction because the chemical character of soil organic matter is important for its environmental persistence, behavior, and turnover dynamics. Soil organic matter content is almost universally reported in basic soil tests, and new soil health analytical packages are now reporting results which target rapid-cycling soil organic matter fractions to track management improvements. There are, however, several different approaches and techniques used to operationally measure soil organic matter, and they are not equivalent. This section of the chapter discusses and compares the common methods of measuring soil organic carbon and soil organic matter and presents considerations to keep in mind when choosing methods and interpreting results.
Table 3.4. Common methods of measuring soil carbon
Method | Measures | Details | Strengths | Disadvantages |
---|---|---|---|---|
Dry combustion | Carbon | Sample combusted in O2 atmosphere | Direct measure of soil C; can be automated; can measure N as well | Carbonates in calcareous soils will confound this measurement |
CO2 evolved is measured directly, usually >1000ºC | ||||
Loss on ignition (LOI) | Organic matter | Sample combusted in O2 atmosphere | Simple equipment | No discrimination of C from other components of organic matter |
Measures mass loss | Bulk analysis | Structural water contributes to mass loss | ||
Usually ~500ºC | ||||
Wet oxidation | Organic matter | Chemically oxidized with chromate (Na2Cr2O7) | Targets organic matter only | Chromate is carcinogenic |
Back-titrated with Fe(II) or measured spectroscopically | Carbonate does not react | Reaction is incomplete | ||
Chromate reacts with other soil components |
Dry Combustion
Dry combustion is a widespread technique used to determine total carbon and nitrogen simultaneously. Air dried and pulverized soil samples (usually <250 μm diameter) are introduced into a combustion furnace at about 1250°C. The samples are combusted in a stream of O2, producing CO2 and N oxide gases (i.e., NOx). The pulse of O2 serves to ensure that all the C and N are oxidized. Following combustion, the gases are swept out of the combustion tube in a flow of helium into a reduction column filled with chopped copper wire. This column is held at 600°C and serves to scavenge O2 from any NOx in the gas stream, thus converting all N to N2. Following the reduction column, the gas stream is dried by passing through a water trap containing magnesium perchlorate. The gas stream is then passed into a packed column gas chromatograph, which separates the CO2 from N2, with the N2 exiting the column first. CO2 and N2 are detected using thermal conductivity detectors or using IR detectors.
Modifications of these methods can include a separate pretreatment to measure any C present in carbonates by neutralization of carbonate with acid and back titration of the excess acid, which is then subtracted from total C to give organic C. Alternatively, carbonates may be destroyed by treatment with dilute acid before C determination. Other laboratories use combustion at 860°C to selectively decompose only organic carbon. Instrumental methods featuring automated versions of DC methods are now routinely used to determine total C and organic C in many laboratories.
Loss on Ignition
The Loss on Ignition (LOI) is a measure of the change in weight as a result of heating to drive off volatiles and/or remove components by thermal decomposition and/or burning in an oxygen-rich atmosphere. At any temperature, the LOI will be the sum of several processes, including:
- Loss of moisture adsorbed on to mineral grains (mostly removed by heating at 105°C).
- Loss of structurally-bound water from within mineral structures (mostly removed by heating to between 600 and 1000°C).
- Oxidation of organic matter, generally at ≤ 500°C, releasing CO2, CO and H2O.
- Thermal decomposition of carbonate minerals (e.g. magnesite at 750 to 950°C or calcite at 850 to 950°C) releasing CO2.
- Oxidation of metals (in particular Fe2+) in an oxygen-rich atmosphere adding iron oxides.
Wet Oxidation
Also known as the ‘Walkley Black’ method, this uses chromate to chemically oxidize organic C in the sample. Oxidation is facilitated by heat generated from the addition of sulfuric acid. The excess chromate is determined by titration, and the quantity of carbon that was oxidized is calculated from the amount of chromate reduced. This analytical procedure used to be the standard method for measuring soil organic carbon but suffers from several limitations. There is incomplete oxidation of the carbon present in the soil samples, and a correction factor is needed. However, this correction factor may vary among soil types, which leads to bias in the results. For instance, carbon present as charcoal may not be included, and would lead to underestimates of total soil carbon.
Soil Organic Matter vs Soil Organic Carbon and the Conversion Issue
Soil organic carbon (SOC) and soil organic matter (SOM) are not the same quantity. It has, however, become an automatic practice to convert between SOC and SOM using Equation 3:
(3)
This assumes that carbon molecules comprise 58% of the total mass of soil organic matter. This is based on results reported by Waksman and Stevens (1930), and even they reported that this was a conversion factor that should be regarded with caution. Soil organic matter also contains hydrogen- and oxygen-containing functional groups, which contribute to the overall soil organic matter mass. While it also is assumed that hydrogen contributes ca. 4% and oxygen contributes ca. 30% to the overall composition, we know that this depends on the decomposition state of soil organic matter. Indeed, hydrogen and oxygen indices that were originally applied to petroleum characterization (Lafargue et al., 1998) can also be used to infer decomposition status in soils (Gregorich et al., 2015; Sebag et al., 2006). Soil organic matter is also an important store of macro-elements crucial in plant nutrition (i.e., N, P, and S). N, P, and S are usually present in relatively low concentrations in soil organic matter (<5% for N, <2% for P and S). A critical review of the commonly-used conversion factor (Pribryl, 2010) and showed that the majority of studies through the years report that this conversion ought to be higher. Figure 3.7 shows that actual relationships between soil organic matter and soil organic carbon vary significantly from the standardized correction, and typically favour higher conversion factors (i.e., lower proportion of C) in soil organic matter.
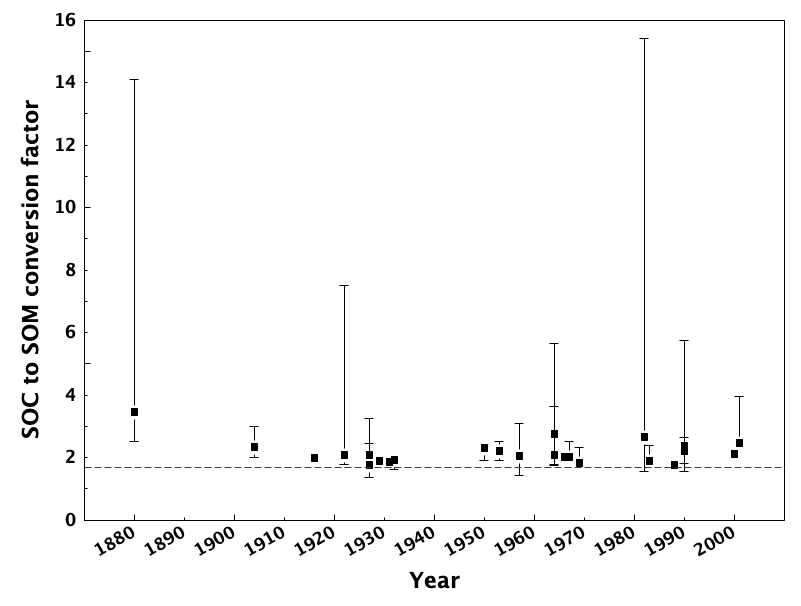
The most effective approach to converting between soil organic matter and soil organic carbon would be to conduct separate direct analyses. Otherwise, be sure to clarify which values were analyzed, and how conversions were carried out.
The Carbonate Issue
Paleozoic carbonate-based (limestone) bedrock is common in many agricultural regions in Canada, mostly in the Prairie provinces and along the Great Lakes and St Lawrence river lowlands. In some areas, particularly those experiencing tillage erosion, carbonates are present in surface soils as well as in parent material horizons. Problems arise when quantifying soil organic carbon in soils because combustion-based analytical methods will decompose carbonates to CO2, thus inflating the overall estimate of organic carbon. Three main approaches are used to differentiate carbonate-C from organic C: heat treatment; acid treatment and; wet oxidation (Table 3.5).
Table 3.5. Methods for sample pretreatment to account for inorganic C (i.e., carbonates) when analyzing soils for total or organic C
Method | Specifics | Benefits | Disadvantages | |
---|---|---|---|---|
Heat Treatment | Indirect organic carbon measurement | Simple, bulk processing possible | Some carbonates decompose at lower temperatures | |
Muffle furnace at 500ºC | Some soil organic carbon resistant at 500ºC | |||
Calculate soil organic carbon by difference | ||||
Acid treatment | Acidify with HCl | Simple, bulk processing | Reduced lifetime of C analyzer | |
Acidify with H2SO3 (sulfurous acid) | Safer for analysis | Requires special evaporative apparatus | ||
Wet oxidation | Redox with chromate to target only organic carbon | Targets organic carbon only | Chromate is carcinogenic | |
Redox reaction with other soil components |
Heat treatment is used in many commercial labs. In this scenario, the soil sample is split into two subsamples. The first half is measured directly by combustion (see Dry Combustion section) giving a measure of total carbon for that soil. The second half is placed in a muffle furnace at ca. 500°C, and this removes organic carbon only, leaving behind the inorganic C. This sample is then measured by combustion, and the organic C is calculated as the difference between the two values. This approach has merit because the common carbonate minerals in Canadian soils have decomposition temperatures above 500°C (i.e., calcite > 700°C, dolomite > 650°C). There are some soils, however, which contain siderite with a lower decomposition temperature around 440°C.
Acid treatment is frequently used because it is inexpensive and does not require specialized apparatus. Acid decomposes carbonates to water and CO2. Two acids are used in laboratory settings: hydrochloric and sulfurous. Hydrochloric acid is relatively easy to work with, but it will reduce the lifetime of carbon analyzers. Sulfurous acid offers the benefit of being volatile, and so evaporates after use. It does, however, require proper ventilation and care in use (Baldock and Skjemstad, 2008).
Wet oxidation, as mentioned above, is a method that targets organic carbon only. The carbonates which may be present in the sample do not participate in the reaction because they already are fully oxidized. This method, however, is based on the use of chromic acids, which are carcinogenic, and may also participate in other redox reactions, thus biasing estimates of organic C.
Soil Organic Matter Composition
In addition to measure soil carbon forms for quantifying soil carbon, soil organic matter composition can also be measured. In many cases, the composition of the soil organic matter is important for better understanding the cycling of carbon, nitrogen and oxygen as well as soil health. Therefore, it is becoming more important to supplement soil carbon measurements with more advanced measures of soil organic matter composition. However, measuring soil organic matter composition is challenging because of the nature of soil organic matter. As reviewed previously in this chapter, soil organic matter is a complex mixture of compounds that are of various sizes, polarity, and stage of oxidation (biodegradation). This continuum of organic compounds makes it challenging to isolate and characterize the composition of soil organic matter which is why in many cases, scientists use multiple techniques that provide atomic or molecular level information (Kögel-Knabner, 2000; Simpson and Simpson, 2012; Derenne and Nguyen Tu, 2014). For this reason, in many cases the soil organic matter is extracted into different fractions. One common and more traditional approach is the fractionation based on solubility in strong base and strong acid, which yields three fractions (fulvic acid, humic acid and humin) that can then be characterized using other techniques. The criticism of this method is that these fractions are not representative of any biogeochemically relevant processes that occur in soil environments (Lehmann and Kleber, 2015). Consequently, another method of fractionating soil organic matter is using density-based methods which separate particulate organic matter (which is not bound to clay minerals) and organic matter associated with different size fractions (for example: sand, silt and clay sized fractions; (Gregorich et al., 1996). Other studies have emphasized the physical fractionation method because it is believed to be more representative of soil organic matter biogeochemical cycling (Mikutta et al., 2006; Sollins et al., 2009; Hatton et al., 2012). For example, the particulate organic matter fraction (also called the light fraction) is fast cycling and accessible to microbes whereas the clay fraction holds soil organic matter that may not be biogeochemically active and is the portion of soil organic matter that is more persistent. Different sized soil aggregates can also be isolated and studied, depending on the research questions or objectives of the analysis.
There are many different advanced and sophisticated techniques that have been developed over the last several decades and applied to study the composition of soil organic matter in whole soils and soil fractions. These methods have improved our understanding of soil organic matter chemistry and persistence in the environment. Methods that use mass spectrometry and nuclear magnetic resonance spectroscopy, two commonly used tools in chemistry, have enabled the understanding of the molecular level composition of soil organic matter (Kögel-Knabner 2000; Simpson and Simpson 2012; Derenne and Nguyen Tu 2014; Simpson et al. 2018). Mass spectrometry techniques are coupled with chromatography methods which separate components before they are analyzed in the mass spectrometer. This has allowed researchers to unravel some of the molecular complexity of soil organic matter and also identify specific compounds within the mixture. Mass spectrometry is also used to measure different stable and radiogenic isotopes of important elements such as carbon, nitrogen, and oxygen. Stable isotopes can provide information about the different plant sources and degree of microbial processing of organic matter (through measuring isotope fractionation (Whalen et al. 2014; Kohl et al. 2015)) whereas radiogenic isotope measurements of carbon (radiocarbon) provides information about the age of organic matter and can be used to better understand the mechanisms of persistence (Trumbore and Druffel 1995; Quideau et al. 2000).

Nuclear magnetic resonance spectroscopy is another method that has provided unparalleled insight into the composition of soil organic matter. Nuclear magnetic resonance spectroscopy can analyze samples in the solid-state or solution-state which is ideal for the analysis of whole soils and various soil fractions (insoluble or soluble) because this technique provides molecular information about all the structures present (Figure 3.8). The Canadian Light Source, at the University of Saskatchewan, is a national research facility where advanced synchrotron light methods can also be used to study soil organic matter composition. These synchrotron light methods can be thought of as powerful x-rays that provide information about the composition of different elements in matter, such as soils. Synchrotron methods can also provide information about interactions between soil organic matter, clays and other materials found in soil (Gillespie et al. 2015). As such, these techniques, as well as others, are rapidly changing our understanding of soil organic matter composition and the underlying mechanisms that control its stability in the environment. Future research endeavours will continue to develop new methods and apply these to better understand the long-term sustainability of soil organic matter in a changing world.
Nuclear magnetic resonance (NMR) spectroscopy has been used for several decades to study the composition of soil organic matter. There are many different types of NMR experiments that can be used (see Simpson et al. 2018 for more detail) but one of the most commonly applied methods in soil science is solid-state 13C NMR (Preston et al. 1997). This technique allows for the analysis of all the carbon forms in soil organic matter in a whole soil sample or even different soil physical fractions. An example 13C NMR spectrum (from Simpson and Simpson (2012) for an Ah horizon from an Orthic Black Chernozem (Malmo Soil Series) is shown in Figure 3.8, along with all of the different structural components that can be identified. The table links these different carbon forms to soil organic matter sources. These structures relate to different plant- and microbial-derived compounds that allows researchers to study soil organic matter sources, turnover, and preservation.
Table 3.6. 13C nuclear magnetic resonance (NMR) chemical shift assignments and relation to soil organic matter structural components shown in the NMR spectrum of the Orthic Black Chernozem (Ah horizon). Chemical shift assignments compiled from: (Baldock et al. 1992; Preston et al. 1997; Salloum et al. 2002; Simpson and Simpson 2012)
Chemical shift range (ppm) | Molecular component | Soil organic matter source |
---|---|---|
0-45 ppm | Unsubstituted alkyl carbon: includes straight chain methylene carbon (30-34 ppm) and terminal methyl groups (15 ppm). Branched methylene carbon is found more downfield (35-45 ppm) | Lipids, cutin, suberin, lignin side chains, amino acids/peptides |
45-65 ppm | Substituted alkyl carbon such as that found in amines (45-46 ppm) and methoxyl groups (56 ppm) | Lipids, lignin |
65-95 ppm | Oxygen substituted carbon, ring carbons in carbohydrates, and carbons in ethers | Cellulose and simple sugars, amino acids/peptides, chitin |
95-110 ppm | Di-oxygen substituted aliphatic carbon and anomeric carbon in carbohydrates (105 ppm) | Cellulose and simple sugars |
110-145 ppm | Aromatic carbon | Black carbon/biochar, lignin and aromatic amino acids |
145-160 ppm | Phenolic carbon | Lignin, suberin |
160-220 ppm | Carboxylic, amide and ester carbon, carbonyl carbon | Lipids (including fatty acids), cutin, suberin, and amino acids/peptides |
SOIL ORGANIC MATTER AND GLOBAL ISSUES
Global Carbon Cycle
Rising atmospheric CO2 levels have brought attention to the important role that soils play in the global carbon cycle. Soils are the largest terrestrial store of carbon, and scientists and policy makers alike have advocated increasing soil carbon sequestration as a way to remove some CO2 from the atmosphere.
Soil carbon stocks are most commonly expressed as kilograms (kg or 103 g), petagrams (Pg or 1015 g). Sometimes, gigatonne (Gt) is used as a unit; note that one Gt = one Pg. Stocks refer to the total amount of carbon contained within a given depth (e.g. the top 30 cm or 50 cm of soil). There are still major uncertainties around the estimates of global soil carbon stocks. Uncertainties are linked to limited data on deep carbon stocks (below 30 cm, to either 1 m or 3 meter depth), as well as to limited data in some geographical areas (such as in northern high-altitude soils). In addition, soil carbon stocks remain hard to model accurately as they depend on the interactive effects of several environmental factors, namely climate, vegetation, and the soil geological parent material.
In 2017, the Food and Agriculture Organization (FAO) of the United Nations launched the Global Soil Organic Carbon Map (GSOCmap) initiative. More than 100 countries contributed to produce the first global soil map ever produced from harmonized national soil maps. This map can be assessed on the GSOCmap portal. Based on these data, the global soil organic carbon stocks for topsoil (0-30cm) represent 680 Petagrams (Pg). Over 70% of these stocks are held by 14 countries, with the largest contributor being the Russian Federation (147.9 Pg – 21.9%), followed by Canada (80.2 Pg – 11.9%), and the United States of America (54.4 Pg – 8.0%).
The majority of global soil carbon stocks lie below 30 cm. Estimates to 1 m average 1,505 Pg, and up to 3,444 Pg of carbon that may be stored between 0 and 3 m. This is more than what the atmosphere and vegetation combined store.
Agricultural activities and land use change account for about 25% of the CO2 anthropogenic emissions. Sequestering additional carbon in soils could help offset these emissions. The “4 per mille initiative- Soils for Food Security and Climate” (also known as the 4 PT- or 4 per thousand) was launched in 2015 during COP21, which stands for the “21st Conference of the Parties” to the United Nations Framework Convention on Climate Change (UNFCCC). This initiative aspires to compensate for the current increase in atmospheric CO2 by increasing global soil carbon stocks by 4 per 1000 (or 0.4 %) per year (see 4per1000). Agriculture and associated land-use change contributes a little under 15% of total anthropogenic CO2 emissions to the atmosphere (Figure. 3.9).
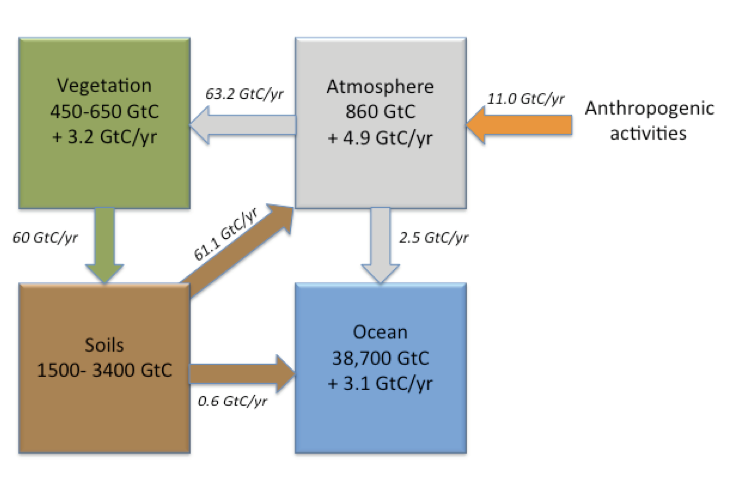
Climate Change and Soil Carbon and Climate Change
Climate change is altering many biogeochemical processes on the planet. Changes in both temperature and moisture have changed the quantity and quality of plant inputs to soil, and have shifted microbial activity, diversity and their subsequent substrate usage (Melillo et al. 2017; Pold et al. 2017; Lajtha et al. 2018). As described earlier, soils are a major reservoir of carbon. These carbon reservoirs are major carbon sinks in the global biogeochemical cycle. However, climate change is shifting some sinks to sources due to the fundamental alteration of processes that regulate soil carbon storage. For example, soil warming experiments have shown that warming can promote microbial activity which then increases soil organic matter degradation and decreases the total amount of soil carbon stored (Melillo et al. 2017). Sensitive ecosystems found in the Arctic are particularly vulnerable because of thawing permafrost which is contributing previously stored carbon into biogeochemically active zones (Schuur et al. 2015). Increases in fire frequency and intensity in the boreal forest are increasing soil carbon losses and threatening the ability of this biome to act as a carbon sink (Walker et al. 2019). Current predictions show a global loss of soil carbon via enhanced respiration across all ecosystems (Bond-Lamberty et al. 2018). Scientists are actively studying how sensitive soil carbon is to climate change impacts in the hopes to determine how to preserve soil carbon stocks. The future of soil carbon remains uncertain and continued soil carbon and soil organic matter losses may result in widespread declines in soil fertility and health. As such, understanding the nature and persistence of soil organic matter and how it is impacted by climate change will result in the development of informed climate change mitigation strategies that preserve and protect soil carbon in the future.
Can You Dig It!
Earthworms- friends or foes?

Beneficial effects of earthworms on soil fertility are numerous. In agricultural soils, they have been reported to increase organic matter, decrease bulk density and increase drainage, and boost crop yield.
In the boreal forest soils of Canada, however, where they are not part of the native biological communities, they are rapidly changing the distribution of soil carbon stocks.
Estimated global carbon stocks in boreal soils range between 367 and 1716 Pg, with a median value at 1095 Pg. About one third of all boreal forests is in Canada. Earthworm invasion results in a reduction or even disappearance of the forest floor, with some of the carbon being transferred to the mineral soil. In the short term, earthworm invasion may result in a net release of carbon to atmosphere. In the longer term, the verdict is still out.
Can You Dig It!
Important Canadians in Soil Organic matter Research
Dr. Caroline Preston, Pacific Forestry Centre, Natural Resources Canada.

Dr. Preston is an expert in soil chemistry and is considered one of the pioneers in applying the 13C-NMR technique in the study of the complex structure of soil organic matter in both agricultural and forest soils. Her research program embodied the following aspects: improving the understanding of the cycling of C, N and P in the soil and use this understanding to enhance the storage of C (climate change implications) and to manage soil N and P and most significantly, pioneering the application of spectroscopic techniques (13C-NMR in particular) in soil science. Among her many important contributions is her work on the chemical structures in forest fire-derived char. Her persistence and understanding of both the methodology of NMR and of soil systems developed an innovative and highly influential body of research which has impacted our understanding of soil organic matter around the world. Dr. Preston received her undergraduate and graduate training in chemistry from McMaster University (1970) and the University of British Columbia (1975), respectively. In 1991, she was elected a fellow of the Chemical Institute of Canada. In 1992, Dr. Preston received the Barringer Award for Applied Spectroscopy from the Spectroscopy Society of Canada and is a fellow of the Canadian Society of Soil Science.
Dr. Morris Schnitzer, Agriculture and Agri-Food Canada

Dr. Schnitzer is a soil chemist who was a pioneer in the study of soil organic matter. His contributions to this field are unparalleled, with his main contributions centering on the analytical methods of extracting, characterizing and modelling humic substances. Many feel he is the grandfather of humic substance study; his publication record includes over 350 refereed articles and many books. He was born to a Jewish family in Germany and fled the Nazis throughout the war. This odyssey has been detailed in his autobiography. He settled in Montreal where he studied soil chemistry and pioneered the early use of gas chromatography, mass spectrometry and NMR to study soil organic matter. For example, in the 1960s, he started a long-term investigation on the oxidative degradation of humic substances and whole soils using very early forms of gas chromatography and mass spectrometry. Never afraid of controversy, he also pioneered the use of pyrolysis mass spectrometry which led to his assertion of the prevalence of N-containing structures in soil organic matter. Dr Schnitzer obtained his B.Sc. with first class honors in 1951, M.Sc in 1952, and Ph.D. in 1955, all from McGill University in Soil Chemistry. He is a fellow of the Canadian Society of Soil Science, Soil Science Society of America, American Society of Agronomy, and Royal Society of Canada.
Dr. Con (Constantine) Campbell, Agriculture and Agri-Food Canada
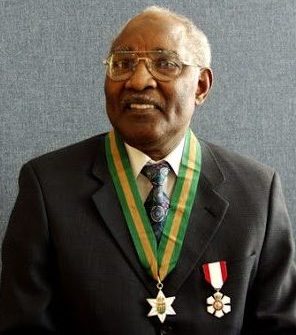
Dr. Con Campbell, is recognized worldwide as one of the foremost specialists in research on soil organic matter and all aspects of nitrogen in soils and crops. Through his research he demonstrated that soil organic matter is a key environmental indicator on health status of prairie soils; his research has received attention nationally and internationally and is used in the development and testing of plant growth models. Con Campbell’s incredible contributions have been recognized; he has been awarded the Order of Canada as well as the Saskatchewan Order of Merit. In his research, he was an early leader in the use of carbon dating to measure the dynamics of soil organic matter turnover. He conducted studies that refined the concept of potentially mineralizable nitrogen, thus providing research basis to optimize fertilizer nitrogen use efficiency on the Canadian prairies. Dr Campbell was born in Montego Bay, Jamaica where he graduated from Cornwall College before obtaining his BSA and MSA in soil science from Ontario Agricultural College of the University of Toronto in 1960 and 1961. He earned his Ph.D. at the University of Saskatchewan in 1965, and immediately joined the Swift Current Research Station in as a research scientist. He is a Fellow of the Canadian Society of Soil Science, the Agricultural Institute of Canada, the Soil Science Society of America, and the American Society of Agronomy.
SUMMARY
- Organic matter is one of the most important attributes of soils. It decreases soil bulk density, increases its water retention, cation exchange capacity, and buffering capacity. Organic matter is central to the biogeochemical cycling of many plant nutrients and helps support the huge biodiversity present in soils.
- Soils contain both organic and inorganic carbon. Several methods exist to measure soil total carbon and soil organic carbon. Organic carbon is the main constituent of soil organic matter, but caution must be applied when converting between soil carbon and organic matter.
- Plant residues are the major substrate for soil organic matter formation. Soil organic matter is composed of these residues at various stages of decomposition, and also contains, in some cases in large amounts, dead faunal and microbial biomass, and products of microbial synthesis.
- Soils store vast amounts of carbon, more than twice as much as the amount of carbon in the atmosphere and terrestrial vegetation combined.
Soil carbon is not evenly distributed in the landscape, but instead will vary as a function of soil forming factors. - The majority of soil carbon in Canada is contained in Cryosols and Organic soils where cold temperature inhibits carbon decomposition losses.
- Initial cultivation of Canadian prairies caused the loss of about a quarter of the carbon contained in these soils. Some of this soil carbon can be restored using conservation agriculture practices, and planting to perennials.
STUDY QUESTIONS
- Where does soil organic matter come from?
- What is the relationship between soil organic matter and major elements (carbon, oxygen and nitrogen)?
- How does soil organic matter contribute to the total soil cation exchange capacity?
- Which environmental factors determine the persistence of soil organic matter in different parts of Canada?
- What happens to plant material (fresh residues) when added to soil? Where does the carbon go?
- Based on some basic redox principles, why are some forms of soil organic matter preferred microbial substrates over others?
- How do we measure soil organic matter content in soil samples?
- If information about the soil organic matter composition is needed, what types of methods could be used? Are these methods easy to perform or do they require advanced equipment or facilities to carry out these measurements?
REFERENCES
Bailey, V. L., Pries, C. H. and Lajtha, K. 2019. What do we know about soil carbon destabilization?. Environ. Res. Lett. 14: 083004. doi: 10.1088/1748-9326/ab2c11.
Baldock, J.A., Oades, J.M., Waters, A.G., Peng, X., Vassallo, A.M., and Wilson, M.A. 1992. Aspects of the chemical structure of soil organic materials as revealed by solid-state 13C NMR spectroscopy. Biogeochemistry 16 : 1–42.
Baldock, J.A. and Skjemstad, J.O. 2000. Role of the soil matrix and minerals in protecting natural organic materials against biological attack. Org. Geochem. 31: 697–710. doi: 10.1016/S0146-6380(00)00049-8.
Bohn, H.L., McNeal, B.L. and O’Connor, G.A. 1985. Soil Chemistry. 2nd edn. New York, Wiley.
Bond-Lamberty, B., Bailey, V.L., Chen, M., Gough, C.M. and Vargas, R. 2018. Globally rising soil heterotrophic respiration over recent decades. Nature 560: 80–83. doi: 10.1038/s41586-018-0358-x.
Cotrufo, M. F., Wallenstein, M.D., Boot, C.M., Denef, K. and Paul, E. 2013. The Microbial Efficiency-Matrix Stabilization (MEMS) framework integrates plant litter decomposition with soil organic matter stabilization: Do labile plant inputs form stable soil organic matter? Glob. Change Biol. 19: 988-995. doi: 10.1111/gcb.12113.
Derenne, S. and Nguyen Tu, T. T. 2014. Characterizing the molecular structure of organic matter from natural environments: An analytical challenge. C. R. Geosci. 346: 53–63. doi: 10.1016/j.crte.2014.02.005.
Feng, X., Simpson, A.J. and Simpson, M.J. 2005. Chemical and mineralogical controls on humic acid sorption to clay mineral surfaces. Org. Geochem. 36: 1553–1566. doi: 10.1016/j.orggeochem.2005.06.008.
Friedlingstein, P, Jones, M W, O’Sullivan, M et al. 2019. Global carbon budget 2019. Earth System Science Data 11: 1783-1838.
Gillespie, A.W., Philips, C.L., Dynes, J.J., Chevrier, D., Regier, T.Z. and Peak, D. 2015. Advances in using soft x-ray spectroscopy for measurement of soil biogeochemical processes. Adv. Agron. 133:1–32. doi: 10.1016/bs.agron.2015.05.003.
Gregorich, E.G., Gillespie, A.W., Beare, M.H., Curtin, D., Sanei, H., Yanni, S.F., 2015. Evaluating biodegradability of soil organic matter by its thermal stability and chemical composition. Soil Biology and Biochemistry 91, 182–191. https://doi.org/10.1016/j.soilbio.2015.08.032
Gregorich, E. G., Monreal, C.M., Schnitzer, M. and Schulten, H.R. 1996. Transformation of plant residues into soil organic matter: Chemical characterization of plant tissue, isolated soil fractions, and whole soils. Soil Sci 161: 680–693. doi: 10.1097/00010694-199610000-00005.
Hatton, P.J., Kleber, M., Zeller, B., Moni, C., Plante, A.f., Townsend, K., Gelhaye, L., Lajtha, K, and Derrien, D. 2012. Transfer of litter-derived N to soil mineral-organic associations: evidence from decadal 15N tracer experiments. Org. Geochem 42: 1489–1501. doi: 10.1016/j.orggeochem.2011.05.002.
Horwath, W.R. and Kuzyakov, Y. 2018. The potential for soils to mitigate climate change through carbon sequestration. Dev. Soil Sci. 35: 61-92. doi: 10.1016/b978-0-444-63865-6.00003-x.
Janzen, H.H., Campbell, C.A., Gregorich, E.G. and Ellert, B.H. 2018. Soil carbon dynamics in canadian agroecosystems. Pages 57-80 in R., Lal, J.M. Kimble, R.F. Follett and B.A. Stewart, eds. Soil Processes and the Carbon Cycle. CRC Press, Boca Raton, Fla. doi: 10.1201/9780203739273.
Jenny, H. 1941. Factors of Soil Formation. McGraw-Hill, New York. doi: 10.1097/00010694-194111000-00009.
Kelleher, B. P. and Simpson, A. J. 2006. Humic substances in soils: Are hey really chemically distinct? Environ. Sci. Technol. 40: 4605–4611. doi: 10.1021/es0608085.
Kleber, M., Sollins, P. and Sutton, R. 2007. A conceptual model of organo-mineral interactions in soils: self-assembly of organic molecular fragments into zonal structures on mineral surfaces. Biogeochemistry 85: 9–24. doi: 10.1007/s10533-007-9103-5.
Kögel-Knabner, I. 2000. Analytical approaches for characterizing soil organic matter. Org. Geochem. 31: 609–625. doi: 10.1016/S0146-6380(00)00042-5.
Kögel-Knabner, I. 2002. The macromolecular organic composition of plant and microbial residues as inputs to soil organic matter. Soil Biol. Biochem. 34: 139–162. doi: 10.1016/S0038-0717(01)00158-4.
Kögel-Knabner, I. and Amelung, W. 2014. Dynamics, chemistry, and preservation of organic matter in soils. pp. 157-215 In H.D. Holland and K.K. Turekian (eds), Treatise on Geochemistry, Second Edition, vol. 12, Elsevier, Oxford. . doi: 10.1016/B978-0-08-095975-7.01012-3.
Kohl, L., Laganiere, J., Edwards, K.A., Billings, S.A., Morrill, P.L. Van Biesen, G. and Ziegler, S.E. 2015. Distinct fungal and bacterial δ13C signatures as potential drivers of increasing δ13C of soil organic matter with depth. Biogeochemistry 124: 13–26. doi: 10.1007/s10533-015-0107-2.
Kuzyakov, Y., Friedel, J. , and Stahr, K. 2000. Review of mechanisms and quantification of priming effects. Soil Biol. Biochem. 32: 1485–1498. doi: 10.1016/S0038-0717(00)00084-5.
Lafargue, E., Marquis, F., Pillot, D., 1998. Rock-Eval 6 applications in hydrocarbon exploration, production, and soil contamination studies. Revue de l’Institut Francais du Petrole 53, 421–437.
Laganière, J., Angers, D. A. and Paré, D. 2010. Carbon accumulation in agricultural soils after afforestation: A meta-analysis. Glob. Change Biol. 16: 439–453. doi: 10.1111/j.1365-2486.2009.01930.x.
Lajtha, K., Bowden, r.D., Crow, S., Fekete, I., Kotroczo, Z., Plante, A., Simpson, M.J. and Nadelhoffer, K.J. 2018. The detrital input and removal treatment (DIRT) network: Insights into soil carbon stabilization. Sci. Total Environ. 640–641: 1112–1120. doi: 10.1016/j.scitotenv.2018.05.388.
Lal, R. 2018. Digging deeper: A holistic perspective of factors affecting soil organic carbon sequestration in agroecosystems. Glob. Change Biol. 24: 3285-3301. doi: 10.1111/gcb.14054.
Lehmann, J. and Kleber, M. 2015. The contentious nature of soil organic matter. Nature 528: 60–68. doi: 10.1038/nature16069.
Mary, B., Clivot, H., Blaszczyk, N., Labreuche, J. and Ferchaud, F. 2020. Soil carbon storage and mineralization rates are affected by carbon inputs rather than physical disturbance: Evidence from a 47-year tillage experiment. Agr. Ecosys. Environ. 299: 106972. doi: 10.1016/j.agee.2020.106972.
Mayer, M. Prescott, C., Abaker, W.E.A., Augusto, L., Cécillon, L., Ferreira, G.W.D., James, J., Jandl, R., Katzensteiner, K., Laclau, J.-P., Laganière, J., Nouvellon, Y.; Paré, D., Stanturf, J.A., ,Vanguelova, E.I. and Vesterdal, L.2020. Influence of forest management activities on soil organic carbon stocks: A knowledge synthesis. Forest Ecol. Manag. 466: 118127. doi: 10.1016/j.foreco.2020.118127.
Melillo, J. M., Frey, s.D., DeAngelis, K.M., Werner, W.J., Bernard, M.J., Bowles, F.P., Pold, G., Knorr, M.A. and Grandy, A.S. 2017. Long-term pattern and magnitude of soil carbon feedback to the climate system in a warming world. Science 358: 101–105. doi: 10.1126/science.aan2874.
Mikutta, R., Kleber, M., Torn, M.S. and Jahn, R. 2006. Stabilization of soil organic matter: Association with minerals or chemical recalcitrance?. Biogeochemistry 77: 25–56. doi: 10.1007/s10533-005-0712-6.
Oades, J.M. 1988. The retention of organic matter in soils. Biogeochemistry 5: 35–70. doi: 10.1007/BF02180317.
Ohlson, M., Dahlberg, B., Okland, T., Brown, K.J. and Havorsen, R. 2009. The charcoal carbon pool in boreal forest soils. Nature Geoscience 2: 692-695. doi: 10.1038/ngeo617.
Pold, G., Grandy, A.S., Melillo, J.M. and DeAngelis, K.M 2017. Changes in substrate availability drive carbon cycle response to chronic warming. Soil Biol. Biochem. 110: 68–78. doi: 10.1016/j.soilbio.2017.03.002.
Prescott, C.E., Frouz, J., Grayston, S.J., Quideau, S.A. and Straker, J. 2019. Rehabilitating forest soils after disturbance. Developments in Soil Science 36: 309-343.doi: 10.1016/b978-0-444-63998-1.00013-6.
Preston, C.M., Trofymow, J.A., Niu, J. and Sayer, B.G. 1997. 13C nuclear magnetic resonance spectroscopy with cross-polarization and magic-angle spinning investigation of the proximate-analysis fractions used to assess litter quality in decomposition studies. Can. J. Bot. 75: 1601–1613. doi: 10.1139/b97-872.
Pribyl, D.W. 2010 A critical review of the conventional SOC to SOM conversion factor. Geoderma. 156:75-83, doi:10.1016/j.geoderma.2010.02.003
Quideau, S.A., Anderson, M.A., Graham R.C., Chadwick, O.A. and Trumbore, S.E. 2000. Soil organic matter processes: characterization by 13C NMR and 14C measurements.Forest Ecol. Manag. 138: 19–27. doi: 10.1016/S0378-1127(00)00409-6.
Ricklefs, R.E. 2008. The Economy Of Nature. W. H. Freeman and Company, New York.
Salloum, M.J., Chefetz, B. and Hatcher, P. G. 2002. Phenanthrene sorption by aliphatic-rich natural organic matter. Environ. Sci. Technol. 36:. doi: 10.1021/es015796w.
Schmidt, M.W., Torn, M.S., Abiven, S., Dittmar, T., Guggenberger, G., Janssens, I.A., Kleber, M., Kögel-Knabner, I., Lehmann, J., Manning, D.A.C.,Nannipieri, P., Rasse, D.P., Weiner, S. and Trumbore, S.E. 2011. Persistence of soil organic matter as an ecosystem property. Nature 478: 49–56. doi: 10.1038/nature10386.
Schuur, E.A.G. McGuire, A.D., Schädel, C., Grosse, G., Harden, J.W., Hayes, D.J., Hugelius, G., Koven, C.D. , Kuhry, P., Lawrence, D.M., Natali, S.M., Olefeldt, D., Romanovsky, V.E., Schaefer, K., Turetsky, M.R. , Treat, C.C and Vonk, J.E. 2015. Climate change and the permafrost carbon feedback. Nature 520: 171–179. doi: 10.1038/nature14338.
Sebag, D., Disnar, J.R., Guillet, B., Di Giovanni, C., Verrecchia, E.P., Durand, A., 2006. Monitoring organic matter dynamics in soil profiles by “Rock-Eval pyrolysis”: Bulk characterization and quantification of degradation. European Journal of Soil Science 57, 344–355.
Shaw, C.H., Banfield, E. and Kurz, W.A. 2008. Stratifying soils into pedogenically similar categories for modeling forest soil carbon. Can. J. Soil Sci. doi: 10.4141/CJSS07099.
Simpson, A.J., Simpson, M.J., Smith, E. and Kelleher, B.P. 2007. Microbially derived inputs to soil organic matter: Are current estimates too low?. Environ. Sci. Technol. 41: 8070-8076. doi: 10.1021/es071217x.
Simpson, A.J., Simpson, M. J. and Soong, R. 2018. Environmental nuclear magnetic resonance spectroscopy: An overview and a primer. Anal. Chem. 90. doi: 10.1021/acs.analchem.7b03241.
Simpson, M. J. and Simpson, A. J. 2012. The chemical ecology of soil organic matter molecular constituents. J. Chem. Ecol. 38:768–784. doi: 10.1007/s10886-012-0122-x.
Six, J., Conant, R.T., Paul, E.A. and Paustain, K. 2002. Stabilization mechanisms of soil organic matter: Implications for C-saturation of soils. Plant Soil 241: 155-176. doi: 10.1023/A:1016125726789.
Sollins, P., Kramer, M.G., Swanson, C., Lajtha, K., Filley, T., Aufdenkampe, A.K., Wagai, R and Bowden, R.D. 2009. Sequential density fractionation across soils of contrasting mineralogy: evidence for both microbial- and mineral-controlled soil organic matter stabilization. Biogeochemistry 96: 209–231. doi: 10.1007/s10533-009-9359-z.
Soucemarianadin, L. N., Quideau. S.A., Wasylishen, R.E. and Munsen, A.D. 2015. Early-season fires in boreal black spruce forests produce pyrogenic carbon with low intrinsic recalcitrance. Ecology 96: 1575–1585.
Tarnocai, C. 2008. The amount of organic carbon in various soil orders and ecological Provinces in Canada. Pages 81-92 in R., Lal, J.M. Kimble, R.F. Follett and B.A. Stewart, eds. Soil Processes and the Carbon Cycle. CRC Press, Boca Raton, Fla.. doi: 10.1201/9780203739273.
Trumbore, S. E. and Druffel, E. R. M. 1995. Carbon isotopes for characterizing sources and turnover of nonliving organic matter. Pages 7-22 in R.G. Zepp and C. Sonntag, eds. Role of Nonliving Organic Matter in the Earth’s Carbon Cycle. John Wiley& Sons Ltd., Chichester..
VandenBygaart, A. J., Gregorich, e.G., Angers, d.A. and Stoklas, U.F. 2004. Uncertainty analysis of soil organic carbon stock change in Canadian cropland from 1991 to 2001. Glob. Change. Biol. 10: 983-994 doi: 10.1111/j.1365-2486.2004.00780.x.
Walker, X. J., Baltzer, J.L., Cumming, s.g., Day, N.J., Ebert, C., Goetz, S., Johnstone, J.F., Potter, S., Rogers, B.M., Schuur, E.A.G, Turetsky, M.R. and Mack, M.C. 2019. Increasing wildfires threaten historic carbon sink of boreal forest soils. Nature 572: 520-531. doi: 10.1038/s41586-019-1474-y.
Whalen, J.K., Gul, S., Poirier, V., Yanni, S.F., Simpson, M.J., Clemente, J.S., Feng, X., Grayston, S.J., Barker, J., Gregorich, E.G., Angers, D.A., Rochette, P., and Janzen, H.H. 2014. Transforming plant carbon into soil carbon: Process-level controls on carbon sequestration. Can. J. Plant Sci. 94. doi: 10.4141/CJPS2013-145.
About The Authors
Sylvie Quideau, Professor, Department of Renewable Resources, Faculty of Agricultural, Life and Environmental Sciences, University of Alberta
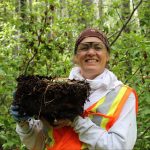
I am a Professor in Soil Biogeochemistry with a special interest in carbon fluxes and organic matter processes. The focus of my research is in three areas: 1) quantifying the underlying environmental factors controlling organic matter accumulation and distribution in soils; 2) relating measurable organic matter quality indices to soil functioning (nutrient fluxes and microbial biodiversity); and 3) predicting the response of soil organic matter to natural and anthropogenic disturbance.
*
Myrna Simpson, Professor and Canada Research Chair (Tier 1) in Integrative Molecular Biogeochemistry, Department of Physical & Environmental Sciences, University of Toronto
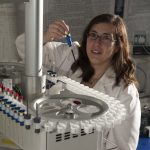
I am a Professor of Environmental Chemistry and Canada Research Chair (Tier 1) in Integrative Molecular Biogeochemistry. I develop and use advanced analytical methods to characterize anthropogenic impacts on soil organic matter composition and dynamics in forests, the High Arctic and agroecosystems. I am also the Associate Director of the Environmental NMR Centre at the University of Toronto Scarborough which is Canada’s only high field NMR facility dedicated to environmental research.
*
Adam Gillespie, Assistant Professor, School of Environmental Sciences, University of Guelph
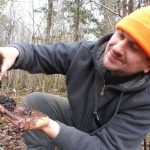
I am an Assistant Professor of Soil Biochemistry in the School of Environmental Sciences. I have a strong interest in using innovative research and instrumentation to link land management with soil health and sustainable land use. In particular, my research focus is on organic matter characterization using spectroscopic and thermal methods, carbon cycling and modelling.
The organic fraction of the soil; includes plant and animal residues at various stages of decomposition, cells and tissues of soil organisms, and substances synthesized by the soil population. It is usually determined on soil that has passed through a 2.0 mm sieve.
The breakdown of a complex material into simpler materials. The complex material can be organic or inorganic, and heat, sunlight, water, chemicals, or metabolism can cause the decomposition. Metabolic decomposition is carried out by decomposer organisms.
A metabolic process in an individual cell, tissue, or organism resulting in the release of chemical energy derived from chemically reduced organic or inorganic nutrients. Specifically, a series of reactions in a cell during which electrons removed during the oxidation of reduced substrates are transferred to an terminal acceptor.
Detachment and movement of soil by water, wind, ice, and/or gravity.
Removal of soluble material from the soil by the downward movement of water.
Arrangement of primary soil particles (sand, silt, clay) around soil organic matter and through particle associations. Soil particles held in a single mass or cluster, such as a clod, crumb, block, or prism. Aggregate stability is a good indicator of soil health.
The total amount amount of exchangeable cations that a soil can adsorb; sometimes called "total exchange capacity," "base exchange capacity," or "cation adsorption capacity". It is expressed in centimoles of charge per kilogram of soil or of other adsorbing material (e.g., clay).
(1) The configuration of a surface area including its relief, or relative elevations, and the position of its natural and manmade features. (2) The study or description of the physical features of the Earth's surface. (3) The physical features of a district or region, such as represented on maps, taken collectively; especially the relief and contour of the land.
The disturbance of sedimentary deposits by living organisms.
(biochemical) The conversion of an organic substance to an inorganic form, often as a result of microbial decomposition. Gross mineralization is the total amount converted, and net mineralization, the gross minus the growth demand or immobilization by the decomposer organisms, usually measured in laboratory incubations.
Not crystalline, or not apparently crystalline. A mineral solid that lacks the long-range order characteristic of a crystal. The internal order of chemical bonds and atoms are either random or very short order. Individual mineral grains are typically very small and produce either no X-ray diffraction pattern or ones with very broad low intensity peaks.
The process of capturing and storing atmospheric carbon dioxide.
Accumulation of organic materials under anaerobic conditions (Organic soils).
Processes involved in the decomposition and partial stabilization of organic matter and leading to the formation of humus.
A process of soil formation resulting in the genesis of Podzolic soils; it involves the translocation of Fe and/or Al organic matter complexes from the A horizon to the B horizon, resulting in the concentration of silica in the layer eluviated.
Reduction of Fe3+ to Fe2+ under anaerobic conditions and transfer of Fe2+.
A system whereby a crop is planted directly into the soil with no primary or secondary tillage since harvest of the previous crop; special seeding equipment is necessary to prepare a narrow, shallow seedbed immediately surrounding the seed being planted. No-till is sometimes practiced in combination with subsoiling to facilitate seeding and early root growth, whereby the surface residue is left virtually undisturbed except for a small slot in the path of the subsoil shank.